the Creative Commons Attribution 4.0 License.
the Creative Commons Attribution 4.0 License.
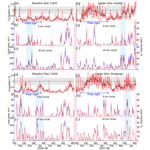
Contrasting two major Arctic coastal polynyas: the role of sea ice in driving diel vertical migrations of zooplankton in the Laptev and Beaufort seas
Igor A. Dmitrenko
Vladislav Petrusevich
Andreas Preußer
Ksenia Kosobokova
Caroline Bouchard
Maxime Geoffroy
Alexander S. Komarov
David G. Babb
Sergei A. Kirillov
David G. Barber
The diel vertical migration (DVM) of zooplankton is one of the largest species migrations to occur globally and is a key driver of regional ecosystems and the marine carbon pump. Previously thought to be hampered by the extreme light regime prevailing in the Arctic Ocean, observations have revealed that DVM does occur in ice-covered Arctic waters and suggest the decline in Arctic sea ice may thereby impact DVM and its role in the Arctic ecosystem. However, coastal polynyas present a unique environment where open water or thin, nearly translucent, ice prevail when offshore winds advect the ice pack away from the coast, allowing light into the surface waters and potentially disrupting DVM. Here, four yearlong time series of acoustic backscatter collected by moored acoustic Doppler current profilers at two opposite sides of the circumpolar polynya system at the Laptev Sea shelf (2007–2008) and the Beaufort Sea shelf (2005–2006) were used to examine the annual cycle of acoustic scattering and therefore the annual cycle of DVM in these areas. The acoustic time series were used along with atmospheric and oceanic reanalysis and satellite data to interpret the results. Our observations show that DVM started to occur once the ice-free surface or under-ice layer irradiance exceeds a certain threshold (from ∼0.3 to 3.3 lx), which is about 2 to 10 times lower in the Beaufort Sea compared to the Laptev Sea. In the Laptev Sea, based on our data and methodology, DVM could not be detected during polar night. In contrast, civil twilight in the Beaufort Sea is sufficient to trigger DVM through polar night. This difference in DVM between the Laptev and Beaufort seas is not entirely assigned to the 3° difference in latitude between the mooring positions as it is also due to the different light threshold required to trigger DVM, different zooplankton communities' composition, and potentially different depths and predation pressures. We find examples in both the Laptev and Beaufort seas where the formation of polynyas and large leads caused DVM to abruptly cease or be disrupted, which we attribute to predator avoidance by the zooplankton in response to higher polar cod (Boreogadus saida) abundance near the open water. Finally, light attenuation by sea ice in the Beaufort Sea caused DVM to extend onto the polar day until the summer solstice. Overall, our results highlight the role of sea ice in disrupting synchronized DVM, the spatial variability in the relationship between sea ice and DVM, and the potential ecological impact of significant trends toward a more extensive circumpolar Arctic coastal polynya as part of changing ice conditions in the Arctic Ocean.
- Article
(16954 KB) - Full-text XML
-
Supplement
(8691 KB) - BibTeX
- EndNote
Every day zooplankton migrates vertically to rise at sunset and to descend with sunrise according to the daily cycle of irradiance. This process is known as diel vertical migration (DVM). DVM is a geographically and taxonomically widespread behavior of zooplankton that spans across diel and seasonal timescales following the latitudinal variation in daylight (Bandara et al., 2021). DVM is arguably the most widespread daily migration of animals on Earth (Hays, 2003) and the largest non-human migration (Brierley, 2014). For the mesopelagic twilight zone, the biological pump and the biogeochemistry is significantly impacted by DVM (e.g., Archibald et al., 2019). The carbon transported by DVM can account for up to 30 % of the total particulate flux and as much as 50 % of the metabolic carbon dioxide produced in the twilight zone (Bianchi et al., 2013).
In all oceans, including the Arctic Ocean, DVM is primarily driven by irradiance (e.g., Hobbs et al., 2021). However, over the past 2 decades DVM was observed to occur during the polar night (Berge et al., 2009, 2015), and more recent studies have analyzed the role of moonlight in modifying DVM during polar night (Last et al., 2016; Petrusevich et al., 2016). Furthermore, oceanographic research using moored acoustic Doppler current profilers (ADCPs) revealed the role of wind-driven and tidal-driven water dynamics in modifying DVM (Petrusevich et al., 2016, 2020; Dmitrenko et al., 2020).
In the Arctic Ocean, the ice cover attenuates light transmission and therefore modifies DVM (Hobbs et al., 2018, 2021; Dmitrenko et al., 2020; Flores et al., 2023). Thereby, over the Arctic and sub-Arctic shelves, which are seasonally covered with perennial sea ice, DVM is also partially controlled by the ice cover (Wallace et al., 2010; Dmitrenko et al., 2021). For this reason, one may assume that small- and large-scale disruptions of the sea ice cover could impact DVM locally and regionally. A thinner sea ice cover in the central Arctic Ocean could thus keep zooplankton deeper for longer (Flores et al., 2023). The seasonal variations in irradiance and ice cover also play a role in triggering the zooplankton seasonal vertical migration (SVM) over the Arctic and sub-Arctic shelves. Beyond irradiance, SVM is also driven by adaptation to seasonality in resources. At high latitudes, an upward migration of herbivorous zooplankton in spring utilizes the high primary production in surface layers during spring bloom, followed by a downward migration in autumn to reside at greater depth during the non-productive autumn and winter (Conover and Huntley, 1991; Conover and Siferd, 1993; Søreide et al., 2022).
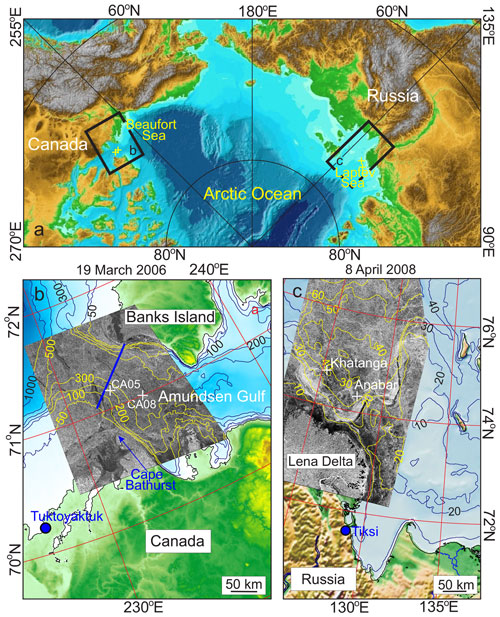
Figure 1(a) Map of the eastern Arctic Ocean adopted from Jakobsson et al. (2000) with positions of the yearlong oceanographic moorings (yellow crosses) deployed in the Beaufort and Laptev seas. Black rectangles show the eastern Beaufort and Laptev seas, as enlarged in (b) and (c), respectively. (b) Map of the eastern Beaufort Sea extended to the Amundsen Gulf with overlaid RADARSAT-1 satellite image from 19 March 2006. Deep blue, white, and yellow lines depict bathymetric contours. Blue line shows conductivity–temperature–depth (CTD) sections sampled in September 2005 and 2006. White crosses mark moorings CA05-05 and CA08-05 deployed from September 2005 to September 2006 in the area of the Cape Bathurst polynya. (c) Map of the southeastern Laptev Sea shelf with an overlaid Envisat advanced synthetic-aperture radar (ASAR) image from 8 April 2008. Deep blue and yellow lines depict bathymetric contours. White crosses mark moorings Khatanga and Anabar deployed from September 2007 to September 2008 in the area of Siberian coastal polynya.
Beyond light, the sea ice disruptions can deviate zooplankton vertical migrations through the polynya-related processes. The persistent regions of open water and thin ice in the Arctic Ocean and the adjoining Arctic shelves called polynyas substantially impact physical and biogeochemical processes in the water column. Polynyas provide access to light. In combination with resuspension of nutrients, this extends the normally short Arctic phytoplankton bloom (Tremblay et al., 2002; Arrigo and van Dijken, 2004; Elias, 2020), attracting zooplankton and fish and providing a rich harvest for marine predators and access to birds and mammals in the otherwise ice-covered water (Arrigo, 2007; Heide-Jørgensen et al., 2013). Over the Arctic shelves, the circumpolar polynya system forms off fixed obstacles comprised of unmovable landfast ice area under offshore wind forcing. Following the inventory of Barber and Massom (2007), north of 68° N the circumpolar Arctic polynya is comprised of 17 individual polynya regions. They are described in detail in Preußer et al. (2016, 2019). Among these 17 polynya regions, the Laptev Sea polynya of the Siberian shelf and the Cape Bathurst polynya of the Beaufort Sea shelf (Fig. 1) appear to be rather comparable in terms of polynya area and accumulated sea ice production. On average, from December to March (2002/2003 to 2017/2018) the Laptev Sea polynya extends over 10.6 ± 4.2 km2, generating 70 ± 28 km3 of new ice (Preußer et al., 2019). The Cape Bathurst polynya is marginally smaller for the same period, extending to 7.5 ± 4.4 km2 and generating 46 ± 26 km3 of new ice (Preußer et al., 2019). The Laptev Sea polynya was extensively studied in 2007–2012 under the framework of the Russian–German project named “The Eurasian Shelf Seas in the Arctic's Changing Environment: Frontal Zones and Polynya Systems in the Laptev Sea” (Kassens, 2012) and during subsequent projects from 2013 to 2021. The Cape Bathurst polynya was assessed in 2007–2008 during the Canadian project named “The Circumpolar Flaw Lead (CFL) system study” (Barber et al., 2010). These two projects provided data and scientific background for assessing the role of polynyas in deviating or even disrupting the DVM seasonal cycle, which is a focus of the present study.
Among all DVM studies in the Arctic shelves, only Dmitrenko et al. (2021) evaluated the role of coastal polynyas in disrupting DVM over the Laptev Sea shelf. They suggested a predator-avoidance behavior of zooplankton conditioned by higher polar cod abundance attracted by the polynya opening. To date there has yet to be a comprehensive analysis of how DVM is modified by the opening of a polynya and what the regional difference in the DVM response to polynya formation is at the eastern and western Arctic shelves. In what follows, we specifically focus on the southeastern Laptev Sea shelf in the eastern (Russian) Arctic and the southeastern Beaufort Sea shelf in the western (Canadian) Arctic. We evaluate the DVM and its modification following the formation of a polynya traced by satellites. Moreover, we quantitatively assess the intensity of acoustic backscatter during polar day and polar night in response to solar irradiation, putting its spatial variability in the context of atmospheric forcing and sea ice conditions.
2.1 Study area
The Laptev Sea shelf represents one of the shallowest and broadest shelf regions of the entire world ocean, extending a distance of ∼500 km offshore (Fig. 1a and c). It is strongly impacted by river runoff. The study area in the eastern Laptev Sea shelf (Fig. 1c) receives an average of 535 km3 of freshwater from the Lena River annually with ∼35 % of the annual discharge occurring in June (Yang et al., 2002). The distribution of the riverine water over the Laptev Sea shelf is entirely wind driven. The cyclonic atmospheric forcing diverges the river plume alongshore eastward, while the anticyclonic wind moves the riverine water offshore northward (Dmitrenko et al., 2005a, 2008, 2010b). The Laptev Sea shelf is seasonally ice-covered from about October to July (Onarheim et al., 2018). Most of the shelf area is covered by landfast sea ice extended approximately to the 20 m depth contour, while mobile first-year pack ice drifts beyond the landfast ice edge. The fast ice reaches a peak thickness in April between 1.5 m further offshore (Belter et al., 2021) and about 2.0 m at the front of the Lena Delta (Hendricks et al., 2018). The interface of the fast ice and pack ice is dynamic; it is frequently covered by a vast latent heat polynya that forms when offshore winds advect the mobile pack ice away from the landfast ice edge (e.g., Dmitrenko et al., 2005b; Willmes et al., 2010, 2011; Kirillov et al., 2013; Gutjahr et al., 2016). The mean ice thickness within the polynya is ∼11 cm, with approximately half of the polynya covered by ice with thickness of 12–20 cm and less than 2 % of the polynya covered by open water and ice thinner than 2 cm (Willmes et al., 2011). The polynyas impact on sea ice production and vertical mixing in the Laptev Sea has been extensively studied (e.g., Dmitrenko et al., 2008, 2009, 2010a, b, 2012; Willmes et al., 2010, 2011; Kirillov et al., 2013; Preußer et al., 2016, 2019).
The zooplankton communities of the Laptev Sea shelf are composed mostly of copepods, with a domination of small-sized (1.0–1.5 mm body length) organisms (Abramova and Tuschling, 2005; Arashkevich et al., 2018). In the coastal regions of the eastern and southeastern Laptev Sea, small-sized brackish-water neritic species tolerant to low salinity dominate (Kosobokova et al., 1998; Pasternak et al., 2022). The western and northeastern Laptev Sea is affected by advection of the oceanic water and hosts larger-sized (2.0–4.5 mm body length) marine species (Calanus spp., Metridia longa) in addition to small brackish-water taxa. In summer, the larger species are present in the regions with depth >50 m (Kosobokova et al., 1998), but by winter they migrate to the deeper regions of the Laptev Sea continental slope. DVM of zooplankton in the shallow Laptev Sea seems to be conducted by copepods Pseudocalanus spp., Acartia spp., and mysids (Dmitrenko et al., 2021), while in the deeper regions (>50 m depth) Calanus spp. and M. longa are known to perform DVM in summer (Arashkevich et al., 2018). The impact of the Laptev Sea polynya on DVM has not been sufficiently assessed. Only recently Dmitrenko et al. (2021) revealed that the DVM disruptions were attributed to polynya opening. However, for the shallow and strongly stratified water column of the Laptev Sea, the comprehensive analysis of the polynya impact on DVM requires further evaluation.
In contrast to the Laptev Sea shelf, the Beaufort Sea has a narrow continental shelf <110 km wide with waters as deep as 3800 m farther offshore in the Canada Basin (Sharma, 1979; Carmack and MacDonald, 2002; Fig. 1a and b). The Mackenzie River, Canada's longest and largest river, provides the greatest Western Hemisphere discharge to the Arctic Ocean (Rood et al., 2017), transporting an average of 325 km3 of freshwater per year through the Beaufort Sea shelf (Yang et al., 2015). During May–June, the Mackenzie River flow exceeds that for January–April by about 4 times (Yang et al., 2015). Wind forcing controls the diversion of the river plume over the Beaufort Sea shelf (Mulligan et al., 2010; Mol et al., 2018; Mulligan and Perrie, 2019). The alongshore easterly (anticyclonic) wind diverges the Mackenzie River riverine water westward and also favors upwelling of the Pacific- and Atlantic-derived Arctic water onto the Beaufort Sea shelf. In contrast, the alongshore westerly (cyclonic) wind forces eastward transport of the Mackenzie River plume and generates downwelling and storm surges along the Beaufort Sea coast (Carmack and Macdonald, 2002; Dmitrenko et al., 2016, 2018). Freeze-up in the eastern Beaufort Sea begins in October and November, and break-up begins in May (Smith and Rigby, 1981; Johnson and Eicken, 2016; Onarheim et al., 2018). The Cape Bathurst polynya forms in the southeastern Beaufort Sea between Cape Bathurst and Banks Island extending to the Amundsen Gulf (Stirling, 1980; Smith and Rigby, 1981; Barber and Hanesiak, 2004; Fig. 1b). The polynya can be open any time when an ice cover is present but starts becoming prominent in April (Smith and Rigby, 1981). Landfast ice in this area extends offshore to about the 20 m isobath, beyond which the polynya is bounded to the north by drifting pack involved to the Beaufort Sea Gyre (Carmack and MacDonald, 2002). In general, the Cape Bathurst polynya is a consequence of the Beaufort Sea Gyre acting like an ice bridge (Barber and Hanesiak, 2004) and creating conditions conducive to oceanic upwelling recurrently observed in the area (Williams and Carmack, 2008; Sévigny al., 2015).
In the Beaufort Sea, both zooplankton and polar cod (Boreogadus saida), also called Arctic cod in North America, conduct DVM from August until May, but the synchronized DVM stops during polar day (Geoffroy et al., 2016; Dimitrenko et al., 2020). The zooplankton communities of the Beaufort Sea are characterized by different species composition and quantitative structure compared to the communities of the shallow Laptev Sea shelf. In contrast to the Laptev Sea, the larger-sized Arctic oceanic taxa prevail in the Beaufort Sea (Darnis et al., 2022; Darnis and Fortier, 2014). Among these species, the most active diel migrators are the copepods Metridia longa, late copepodites of Calanus glacialis, and chaetognaths Parasagitta elegans, all falling within the size range 2.0–20 mm, a size that is easily detectable by acoustic backscatter. Most copepods also conduct SVM, with large herbivorous copepods such as Calanus hyperboreus and Calanus glacialis entering diapause and descending to the deep Atlantic waters for overwintering and ascending from depths to the surface layers in spring. The smaller zooplankton, the omnivorous copepods Oithona similis and Pseudocalanus spp., occupy intermediate depths continuing to feed and presumably to reproduce during the winter season (Darnis and Fortier, 2012, 2014). Most fish and zooplankton avoid the colder polar mixed layer at the surface year-round (Geoffroy et al., 2011; Darnis and Fortier, 2014). Polar cod also perform SVM towards deep embayment during the winter, presumably for spawning (Benoit et al., 2010; Geoffroy et al., 2011). To our knowledge, the impact of the Cape Bathurst polynya on DVM of fish and zooplankton has never been assessed.
2.2 Observations and data
Here we provide analysis of DVM at two opposite sides of the circumpolar polynya system (Fig. 1a) at the eastern Beaufort Sea shelf (Canadian Arctic; Fig. 1b) and the Laptev Sea shelf (Russian Arctic; Fig. 1c) and give examples of how the opening of a polynya and/or leads affects DVM. Observing DVM in ice-covered waters is difficult; however, moored acoustic Doppler current profilers (ADCPs) provide a time series of acoustic backscatter in the water column that can be used to trace DVM year-round. Oceanographic moorings, equipped with ADCPs, have been deployed annually over the Laptev and eastern Beaufort Sea shelves since the end of 1990s. While ADCPs provided valuable information on physical oceanography, this dataset was rarely assessed to analyze biological backscatter and vertical migrations of zooplankton. Here, we use the ADCP-derived time series of acoustic backscatter from two yearlong mooring arrays (Table 1) deployed in the southeastern Beaufort Sea in September 2005 (moorings CA05 and CA08; Fig. 1b) and in the southeastern Laptev Sea in September 2007 (moorings Anabar and Khatanga; Fig. 1c) to examine polynya impact on DVM. All four moorings were located above the Arctic Circle at 66.6° N (Table 1) at about 74.5° N (Laptev Sea) and 71° N (the Beaufort Sea), the area where the sun is between 6 and 12° below the horizon all day on the winter solstice. At these latitudes, during the nautical polar night period, there is no trace of daylight, only around midday is there is slight light because of refraction, and the horizon and the brighter stars are visible during clear sky. During polar day around the summer solstice, the sun does not move below the horizon, and daylight lasts from sunset to sunrise.
Observations of DVM were derived from four upward-looking ADCPs deployed on bottom-anchored oceanographic moorings designed as an anchored taut line with subsurface flotations and acoustic releases. More details on these moorings can be found in Table 1, and the ADCP data are available in Dmitrenko (2024). The ADCPs were 300 kHz upward-looking Workhorse Sentinel ADCPs from Teledyne RD Instruments. For this study, we used only the acoustic backscatter intensity data that were obtained at intervals of 1 m in the Laptev Sea and 8 m in the Beaufort Sea, with a 30 and 20 min ensemble time interval and 30 and 20 pings per ensemble for the Laptev and Beaufort seas, respectively. Calibration of ADCP acoustic backscatter data was not conducted, so the volume backscatter represents only relative measurements. ADCPs sampled the overlying water column, except the shadow zone over the acoustic transducer and the subsurface water layer, where the acoustic reflection from the air–water and/or ice–water interface compromises the data (Table 1). In the shallow Laptev Sea, nearly the entire water column has been sampled, while in the Beaufort Sea, ADCPs sampled only the upper portion of the water column from 9–10 m to 65 and 82 m depth for CA05 and CA08, respectively (Table 1).
The ADCP-derived vertical velocity has also been used to study rates of zooplankton DVM in the Beaufort Sea (e.g., Plueddemann and Pinkel, 1989; Cottier et al., 2006; Ochoa et al., 2013). The accuracy of the ADCP vertical velocity measurements is not validated; however, the manufacturer reports that the vertical velocity is more accurate than the horizontal velocity by at least a factor of 2 (Wood and Gartner, 2010) and has an accuracy of ±1.0 %. Nevertheless, vertical velocities in the 3 mm s−1 range may well be within the noise level (Miller, 2003).
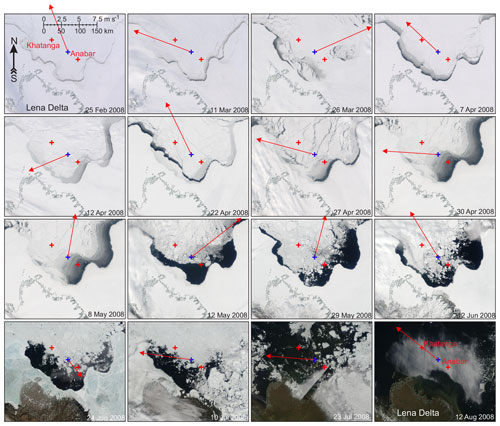
Figure 2The MODIS true-color satellite imagery for February to August 2008 showing the evolution of ice conditions in a relatively cloud-free area of Siberian coastal polynya in the vicinity of the Lena Delta (Laptev Sea). Red crosses mark the Khatanga and Anabar moorings. Blue crosses depict the spatial node where 2 m air temperature and 10 m wind were derived from ERA5 (Fig. S2a and b, respectively). Red arrows show daily mean wind for the day the image was taken.
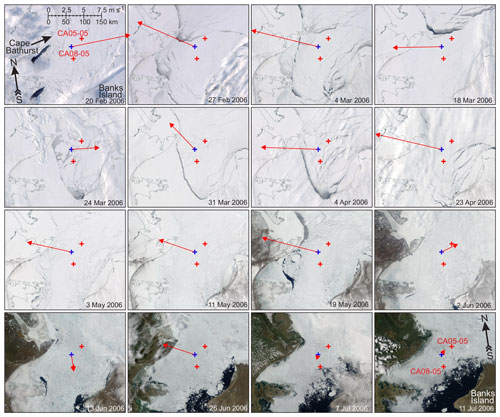
Figure 3The MODIS true-color satellite imagery for February to July 2006 showing the evolution of ice conditions in a relatively cloud-free area of Cape Bathurst polynya in the Amundsen Gulf (Beaufort Sea). Red crosses mark moorings CA05-05 and CA08-05. Blue crosses depict the spatial node where 2 m air temperature and 10 m wind were derived from ERA5 (Fig. S2c and d, respectively). Red arrows show daily mean wind for the day the image was taken.
Fields of sea level pressure (SLP; Fig. S1 in the Supplement), 10 m wind velocity, and 2 m air temperature at 6 h intervals (Fig. S2) were retrieved from the ERA5 atmospheric reanalysis (Hersbach et al., 2020). The horizontal resolution of ERA5 is 31 km. The daily mean cloud fraction data (the percentage of Earth's surface covered by clouds) were derived from the Aqua Moderate Resolution Imaging Spectroradiometer (MODIS) satellite imagery (Platnick et al., 2017). The sensor and algorithm resolution is 5 km, imagery resolution is 2 km, and the temporal resolution is daily. For both the ERA5 and cloud fraction we used data from the grid point closest to the mooring positions in our analysis.
The extent and evolution of the sea ice cover around the moorings between September 2005 and July 2006 in the Beaufort Sea and between September 2007 and September 2008 in the Laptev Sea were analyzed in synthetic-aperture radar (SAR) images collected by RADARSAT-1 and Envisat, respectively. Within the radar images, areas of higher radar backscatter (gray and white in Fig. 1b and c) indicate the formation of new ice in the polynya, while lower radar backscatter (black and gray) indicates open water within the coastal polynya and leads in the surrounding ice pack. The fast ice edge separates the landfast ice from the coastal polynya and is usually evident in the radar images (e.g., Fig. 1c). For cloud-free conditions, when the sun is above the horizon, we also used MODIS true-color satellite imagery from NASA (https://modis.gsfc.nasa.gov, last access: 11 December 2024; Figs. 2 and 3). MODIS imagery spatial resolution is 250 m, and the temporal resolution is daily.
Monthly gridded fields of sea ice concentration around the mooring positions were retrieved from the National Snow and Ice Data Center (Walsh et al., 2019). The ice concentration dataset at 25 km resolution was produced by the NASA Team algorithm (Comiso et al., 1997) and applied to passive microwave brightness temperatures (Cavalieri et al., 1996). Observations of snow depth are from the Advanced Microwave Scanning Radiometer – Earth Observing System (AMSR-E) at 12.5 km spatial resolution (Cavalieri et al., 2014). Snow depth over sea ice is a running 5 d average based on the current day and the 4 previous days.
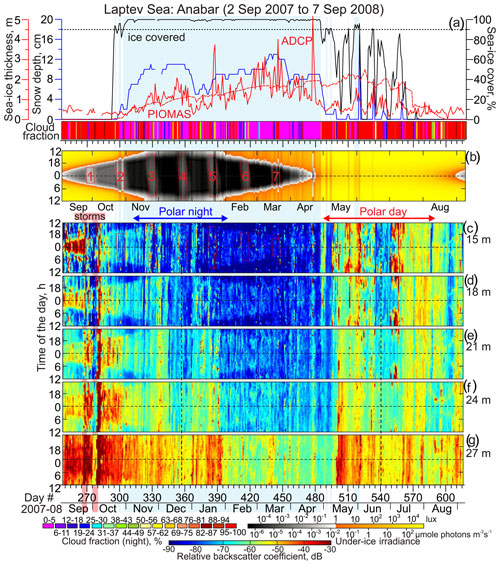
Figure 4Observations at Anabar. Blue shading highlights sea ice cover exceeding 90 %. (a) Time series of the snow depth (cm, blue), sea ice thickness (m, red) and concentration (%, black), and nighttime cloud fraction (shown in color, %). Sea ice thickness is from ADCP (solid red line) and PIOMAS (dashed red line). Actograms of the (b) modeled under-ice irradiance (lx) and (c–g) mean volume backscatter strength (MVBS; dB) at five depth levels: (c) 15, (d) 18, (e) 21, (f) 24, and (g) 27 m depth. (b) Dotted red rectangles with reference numbers depict the full moon occurrences. (c–g) Red and blue arrows at the top indicate the periods of polar day and polar night, respectively. Vertical black lines depict solstices. Pink shading highlights two storms following Hölemann et al. (2011).
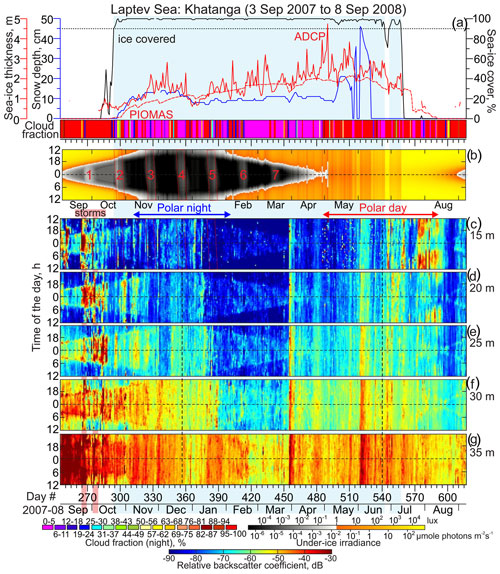
Figure 5Observations at Khatanga. Blue shading highlights sea ice cover exceeding 90 %. (a) Time series of the snow depth (cm, blue), sea ice thickness (m, red) and concentration (%, black), and nighttime cloud fraction (shown in color, %). Actograms of the (b) modeled under-ice irradiance (lx) and (c–g) mean volume backscatter strength (MVBS; dB) at five depth levels: (c) 15, (d) 20, (e) 25, (f) 30, and (g) 35 m depth. All other designations are similar to those in Fig. 4.
During the time of mooring deployment, the spatial and temporal coverage of sea ice thickness observations from spaceborne satellite altimeters was insufficient. Hence, in our analysis we use modeled daily estimates of sea ice thickness around the mooring positions from the Pan-Arctic Ice Ocean Modeling and Assimilation System (PIOMAS; Figs. 4–7a). PIOMAS is a coupled ocean and sea ice model that assimilates daily sea ice concentration and sea surface temperature satellite products (Zhang and Rothrock, 2003). In general, PIOMAS tends to overestimate the thickness of thin ice and underestimate the thickness of thick ice (Schweiger et al., 2011). For the eastern Laptev Sea shelf, from 2003 to 2008 PIOMAS overestimated the thickness of ice relative to ICESat observations by 0.2–0.7 m (Schweiger et al., 2011). This uncertainty can generate significant bias in estimated under-ice irradiance during winter. To better constrain the uncertainty in ice thickness, we use a record of daily mean sea ice draft derived from the bottom-tracking mode of the ADCPs deployed in the Laptev Sea (Belter et al., 2020). However, this approach also has its uncertainties, as comparing ice drafts derived by ADCP and upward-looking sonars (ULSs) reveals the ADCPs underestimate the mean and median thickness by approximately 0.10 to 0.37 m (Figs. 4a and 5a).
For a more precise analysis of the impacts of polynyas and leads on DVM, we used a lead dataset retrieved from MODIS thermal infrared imagery. Reiser et al. (2020) use ice surface temperature (IST) swath data to retrieve a lead data product that features pan-Arctic and quasi-daily categorical maps of leads (with accompanying lead score), artifacts, clouds, and sea ice for the period from 2002 to 2020 (November to April). Thereby, dedicated image-processing strategies allow us to filter out unidentified cloud artifacts using multiple lead metrics in a fuzzy-logic approach (Willmes et al., 2015, 2016). Preußer et al. (2022) indicated that these classified leads often comprise ice thicknesses beyond 20 cm, a commonly used thickness threshold for polynyas and leads. In order to derive parameters that describe the lead activity in proximity of the mooring locations, the MxD29 (MxD indicates MOD29 from Terra and MYD29 from Aqua) Collection 6 sea ice product (Hall et al., 2004; Riggs and Hall, 2015) served as the fundamental data basis. It contains IST calculated from MODIS thermal infrared satellite data at a nominal geometric resolution of 1 km2 at nadir. The MODIS cloud mask (MxD35, Ackerman et al., 2010) acts as a first cloud filter as part of the MxD29 product, with known difficulties in detecting low clouds and sea smoke during winter.
Complementary conductivity–temperature–depth (CTD) profiles were collected at each mooring location during deployment and recovery (Fig. S3) and over the entire area at the front of the Lena River delta in the Laptev Sea (Transdrift, 2007, 2008; Fig. S4) and across the entrance to Amundsen Gulf in the Beaufort Sea (Gratton et al., 2014a, b; Fig. S5). In the Laptev Sea, CTD profiles were collected with a CTD SBE 19plus probe, while a CTD SBE 911plus probe was used in the Beaufort Sea. According to the manufacturer estimates, individual conductivity measurements are accurate to 0.0003 and 0.0005 Sm−1, respectively, for the SBE 911plus and SBE 19plus. Individual temperature measurements for the two types of probe are accurate to 0.001 and 0.005 °C, respectively. The CTD instruments were calibrated by the manufacturer before each expedition.
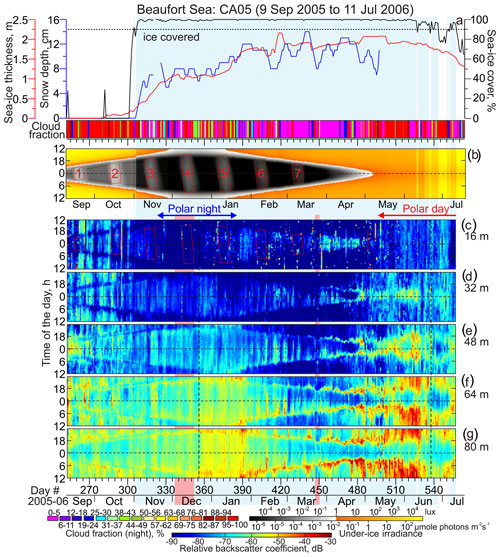
Figure 6Observations at CA05. Blue shading highlights sea ice cover exceeding 90 %. (a) Time series of the snow depth (cm, blue), sea ice thickness (m, red) and concentration (%, black), and nighttime cloud fraction (shown in color, %). Sea ice thickness is from PIOMAS (red line). Actograms of the (b) modeled under-ice irradiance (lx) and (c–g) mean volume backscatter strength (MVBS; dB) at five depth levels: (c) 15, (d) 31, (e) 47, (f) 55, and (g) 63 m depth. Pink shading highlights the lead event in December 2005 and March 2006 (see Figs. 13 and 3, respectively). All other designations are similar to those in Fig. 4.
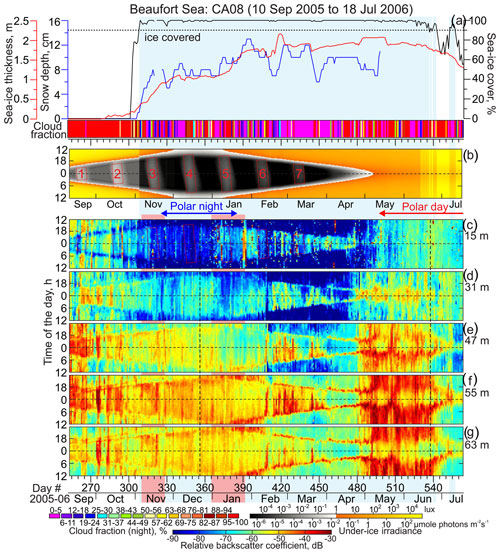
Figure 7Observations at CA08. Blue shading highlights sea ice cover exceeding 90 %. (a) Time series of the snow depth (cm, blue), sea ice thickness (m, red) and concentration (%, black), and nighttime cloud fraction (shown in color, %). Sea ice thickness is from PIOMAS (red line). Actograms of the (b) modeled under-ice irradiance (lx) and (c–g) mean volume backscatter strength (MVBS; dB) at five depth levels: (c) 15, (d) 31, (e) 47, (f) 55, and (g) 63 m depth. Pink shading highlights the lead events in November 2005 and January 2006 (see Fig. 13). All other designations are similar to those in Fig. 4.
2.3 Methods
The methodology used in this study is largely based on that applied by Petrusevich et al. (2016, 2020) and Dmitrenko et al. (2020, 2021). We analyzed the yearlong time series of acoustic backscatter measured by the ADCPs to reveal modifications of the diurnal signal that are the result of DVM. In general, acoustic backscatter is explained by either suspended sediments (e.g., Wegner et al., 2005) or planktonic organisms (e.g., Petrusevich et al., 2020). Frazil ice crystals, which form during new ice growth within the coastal polynyas, also generate an enhanced acoustic backscatter (e.g., Dmitrenko et al., 2010a). However, because of DVM, the acoustic backscatter produced by zooplankton is more complex and easily recognizable from the backscatter generated by sediment particles and frazil ice crystals (Stanton et al., 1994). Moreover, ADCPs, unlike echo sounders, are limited in deriving accurate quantitative estimates of zooplankton biomass (Lemon et al., 2001, 2008; Vestheim et al., 2014). This is mainly due to calibration issues (e.g., Lorke et al., 2004) and the beam geometry (Vestheim et al., 2014). Instead of absolute abundance values, we therefore work with the mean volume backscatter strength (MVBS) in decibels (dB) from the acoustic backscatter echo intensity, following the procedure described by Deines (1999) and updated by Mullison (2017). In what follows, we used ADCP acoustic backscatter from below the surface water layer where the MVBS appears to be contaminated by the reflection of the acoustic signal from the air–water and/or ice–water interface.
To quantify the total sky illumination at each site, we used the skylight.m function from the astronomy MATLAB package (Ofek, 2014). The irradiance values were estimated at the ice-free surface or under the ice. Under-ice illumination was modeled using the exponential decay radiative transfer model (Grenfell and Maykut, 1977; Perovich, 1996). Transmittance through the sea ice and snow cover to depth z in the ice was calculated using the following equation: , where i0 is the fraction of the wavelength-integrated incident irradiance transmitted through the top 0.1 m of the surface layer and Kt is the total extinction coefficient in the snow or sea ice cover. The values adopted for the sea ice and snow cover were i0=0.63 and Kt=1.5 and i0=50.9 and Kt=0.1, respectively (Grenfell and Maykut, 1977). For computing under-ice irradiance in the Laptev Sea (Figs. 4b and 5b), we used ADCP-derived estimates of sea ice thickness from Belter et al. (2020). In the Beaufort Sea, we used PIOMAS-derived thickness. In both cases, snow depth came from AMSR-E. We only accounted for the sea ice and snow cover if the sea ice concentration exceeded 90 %. This implies that if sea ice concentration was less than 90 %, the sea ice cover was not accounted for when light transmission was calculated. Due to a high uncertainty in cloud cover data retrieved from the Aqua/MODIS at high latitudes (Khanal and Wang, 2018), this model does not account for the variation in solar irradiance from clouds.
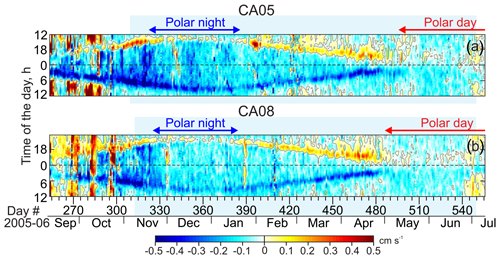
Figure 8Actograms of ADCP-measured vertical velocity (cm s−1) for (a) CA05 and (b) CA08 averaged for 39 to 63 m depth. Positive (negative) values correspond to the upward (downward) flow. Blue shading highlights sea ice concentrations exceeding 90 %. Red and blue arrows at the top indicate the periods of polar day and polar night, respectively.
Time series of MVBS (Figs. 4–7c–g) and surface layer modeled irradiance (Figs. 4–7b), computed from the PIOMAS and ADCP estimates of sea ice thickness and snow depth from AMSR-E, are presented in the form of actograms. Variations during a daylong period are presented along the vertical axis of the actogram, while the long-term patterns of diurnal behavior can be assessed following the horizontal axis (e.g., Leise et al., 2013; Last et al., 2016; Petrusevich et al., 2016, 2020; Hobbs et al., 2018; Dmitrenko et al., 2020, 2021). For the actograms of irradiance, we introduced an artificial visual boundary on the irradiance color scheme at 1 lx (gray to orange), which is the threshold that corresponds to irradiance during the deep twilight. In addition, the vertical velocity actograms were calculated in the Beaufort Sea for the same depths as MVBS actograms and were averaged through the subsurface water layer (Fig. 8). Positive velocities are associated with the upward movement of particles.
In this study, we derive the total lead area (LA) from the MODIS lead product within a predefined reference circle (radii R of 5 and 15 km) around a given mooring location (Anabar, Khatanga, CA05, CA08; Table 1). LA is given as the aerial sum of all pixels that were classified as a lead (i.e., not including artifacts). The corresponding lead fraction (LF) is then the relative proportion of the daily LA with respect to the total area comprised by each circle (i.e., 78.54 km2 for r=5 km and 706.86 km2 for r=15 km). Overall, both metrics serve as an indicator for the presence of thin ice and open water in proximity of the moorings during winter.
Annual actograms of the modeled under-ice irradiance and MVBS were computed for various depths at both the Laptev Sea (Figs. 4 and 5) and the Beaufort Sea (Figs. 6 and 7) based on total sky irradiance, sea ice concentration, sea ice thickness, and snow depth. These actograms reveal a rhythm of activity with the diurnal cycle seen in the vertical axis and the yearlong variability of the diurnal cycle observed along the horizontal axis. The strength of acoustic backscatter is presented by colored contours and provides qualitative information on the concentration of scatters in the water column.
3.1 Light intensity triggering DVM
The MVBS diurnal signal follows the seasonal variability of the sun irradiance with the onset of the descent and ascent following sunrise and sunset, respectively. Actograms in Figs. 4–7 show that there is a level of light intensity that triggers DVM during the twilight period for both the Laptev Sea and Beaufort Sea. The DVM starts when the light intensity during twilight exceeds the light threshold. In general, DVM stops when light intensity is above or below the light threshold 24 h a day (Figs. 4–7). For the Laptev Sea, visual qualitative inspection of the irradiance and MVBS actograms reveals that the light intensity at the surface triggering DVM during the twilight period is ∼1 lx (Figs. 4 and 5), which is equivalent to about W m−2 and µmol photons m−2 s−1. The light intensity of 1 lx corresponds to irradiance shortly after the end of civil twilight (Gaston et al., 2014). This result is consistent with that revealed for the Laptev Sea by Dmitrenko et al. (2021). For the Beaufort Sea, comparing actograms of MVBS and light shows the light intensity triggering DVM of ∼0.1 lx (Figs. 6 and 7) that corresponds to the sun irradiance shortly before the end of the nautical twilight or to the moonlight at full moon with a moon altitude on a clear night (Gaston et al., 2014). This light threshold resembles that obtained for the Beaufort Sea upper continental slope by Dmitrenko et al. (2020).
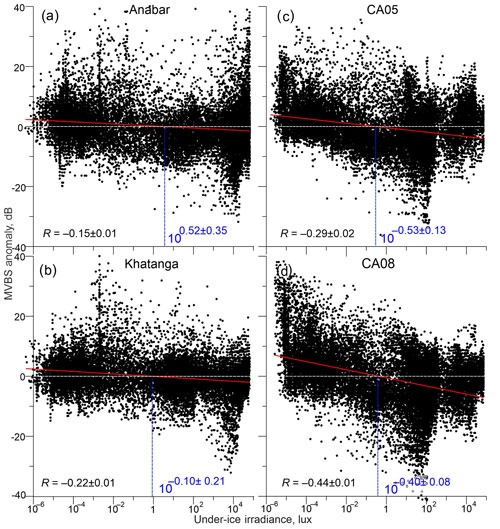
Figure 9Scatter plots show MVBS anomaly (dB) relative to the 7 d running mean for the ADCP uppermost level as function of modeled under-ice irradiance (in a decimal logarithm scale) for (a) Anabar, (b) Khatanga, (c) CA05, and (d) CA08. Red lines show linear regression. Blue numbers show estimates of under-ice irradiance (lx) triggering DVM. It is derived from the linear regression at the zero MVBS anomaly with confidence intervals computed for the 95 % confidence level. R is a linear correlation between MVBS anomaly and under-ice irradiance. Confidence intervals are computed for the 95 % confidence level.
More precise quantitative estimates of the light threshold for triggering DVM are conducted based on the linear regression between simulated light irradiance as the independent variable (Figs. 4–7b) and MVBS as the dependent variable (Figs. 4–7c). The dependent variable (MVBS from the ADCP uppermost level) was preliminary filtered with a 7 d running mean to retain the true signal representing DVM. We suggest that the light threshold for triggering DVM corresponds to the zero anomaly of the filtered MVBS, which is derived from the linear regression (Fig. 9). All statistical estimates are provided for the 95 % confidence level.
Our analysis shows statistically significant negative correlations between light and MVBS; however, the correlation coefficients for the Laptev Sea ( and for Anabar and Khatanga, respectively) are significantly lower than those for the Beaufort Sea ( and for CA05 and CA08, respectively). Note that all the correlations are statistically significant. This difference is explained by two factors, as we discuss in detail in Sect. 3.2 and 3.4: (i) there is no DVM in the Laptev Sea during polar night, and (ii) DVM in the Laptev Sea is impacted by polynya to a greater extent compared to the Beaufort Sea.
The linear regression analysis confirms different light thresholds triggering DVM in the Laptev and Beaufort seas (Fig. 9). In the Laptev Sea, the linear regression reveals the light threshold of 100.52±0.35 and 10 lx that correspond to 3.3 and 0.8 lx for Anabar and Khatanga, respectively (Fig. 9a and b). Taking into account the confidence intervals, the difference between Anabar and Khatanga only slightly exceeds the confidence level because of a high scatter in Fig. 9a and b. For the Beaufort Sea, we estimate the light threshold to 10 and 10 lx that correspond to 0.3 and 0.4 lx for CA05 and CA08, respectively (Fig. 9c and d). The difference between CA05 and CA08 is statistically insignificant. Overall, our results show that the light threshold at the sea surface or below the ice triggering DVM in the Laptev Sea is about 2 to 10 times higher compared to the Beaufort Sea.
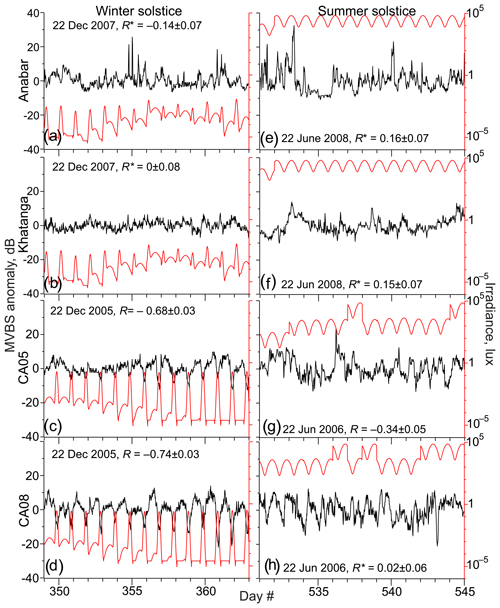
Figure 10Time series of MVBS anomaly (black, dB) relative to the 7 d running mean for the ADCP uppermost level and modeled under-ice irradiance (lx) in a decimal logarithm scale (red) for 7 d before and after winter (left) and summer (right) solstices for (a, e) Anabar, (b, f) Khatanga, (c, g) CA05, and (d, h) CA08. Correlation R between the MVBS anomaly and under-ice irradiance estimated for the 95 % confidence level. Asterisks highlight statistically insignificant correlations.
3.2 Diurnal signal of the mean volume backscatter strength during polar night
During polar night (i.e., when the sun is below the horizon 24 h a day), the DVM stops when the light intensity does not exceed the light threshold through the entire 24 h. This is justified based on the disappearance of the difference in MVBS between midnight and noon. For the Laptev Sea, MVBS data shows that during a majority of polar night there is no difference between acoustic scattering at midnight and noon as soon as under-ice light intensity falls below the light threshold revealed in Sect. 3.1 (Fig. 9a and b). This indicates vanishing of DVM observed through the water column resolved with ADCP data at both Anabar and Khatanga (Figs. 4 and 5). For example, in the Laptev Sea ± 7 d from the winter solstice, through the subsurface water layer there is no correlation between MVBS anomaly and light intensity, which does not exceed 10−2 lx (Fig. 10a and b) This light intensity is far below the light threshold for triggering DVM in the Laptev Sea. In the Beaufort Sea, when under-ice irradiance falls below 0.2 lx during 2 weeks around winter solstice, MVBS maintains a difference between midnight and noon, reducing around noon by ∼10 to 20 dB compared to midnight (Fig. 10c and d). Moreover, correlation between the MVBS anomaly and light intensity through the subsurface water layer is estimated to be −0.68 and −0.74 for CA05 and CA08, respectively (Fig. 10c and d). This indicates the occurrence of DVM during polar night while the sun is below the horizon 24 h a day (Figs. 6 and 7). However, the DVM signal is traceable during the entire polar night only at the upper level down to 31–32 m depth (Figs. 6c and d and 7c and d). For the deeper water layer, the DVM signal disappeared after winter solstice (Figs. 6e–g and 7e–g).
For the Beaufort Sea, another set of actograms was generated for the ADCP-measured vertical velocity averaged for 39 to 63 m depth (Fig. 8). During polar night, vertical velocity actograms show a nearly symmetrical diurnal pattern around astronomic midnight that is consistent with the backscatter actograms. Net upward flow was observed daily before astronomic midnight. Note that in the Laptev Sea actograms for the ADCP-measured vertical velocity did not reveal DVM.
In summary, our data from the Laptev Sea do not indicate DVM is observed during the major portion of polar night. However, small-scale DVM (e.g., Ludvigsen et al., 2018) or DVM by smaller organisms than detected by a 300 kHz ADCP (<1.5 mm) cannot be fully discriminated. In the Beaufort Sea, DVM is conducted throughout the entire polar night. In terms of the light conditions during polar night, the study area in the Beaufort Sea is substantially different from that in the Laptev Sea. In the Beaufort Sea (mooring array at ∼71° N, Table 1), civil twilight (the sun's disk at most 6° below the horizon) is observed through the entire polar night after sunset and before sunrise. Duration of civil twilight ranges from ± 3 h at noon on 22 November 2005 (beginning of polar night at 71° N), to ± 1.33 h on 22 December 2005 (winter solstice), and to ± 2.65 h on 22 January 2006 (end of polar night at ∼71° N). This means that during polar night, civil twilight occurs at ÑA05 and CA08, illuminating the subsurface under-ice water layer even during the winter solstice from ∼0.2 lx at noon to 0.05 lx at the end of civil twilight (Figs. 6b, 7b, 10c, and d). In contrast, in the Laptev Sea at ∼74.5° N (Table 1), there is no civil twilight during the portion of polar night from 30 November 2007 to 13 January 2008 when the sun remains >6° below the horizon. During this time, the light intensity below the ice does not exceed 10−2 lx observed during full moon events (Figs. 6b, 7b, 10a, and b). However, the difference in DVM between the Laptev and Beaufort seas during polar night is not entirely assigned to the different latitudinal position but is also attributed to the different magnitude of the light threshold for triggering DVM (see Sect. 3.1).
3.3 Diurnal signal of the mean volume backscatter strength during polar day
The response of the MVBS diurnal pattern to enhanced irradiance during polar day (the sun is above the horizon 24 h a day) in the Laptev Sea differs from that in the Beaufort Sea. During polar day, the MVBS diurnal rhythm in the Laptev Sea completely vanished (Figs. 4, 5, 10e and f). This is attributed to enhanced sunlight with an increase in the midnight under-ice irradiance to >15 lx from the beginning of May (Figs. 4b and 5b) to ∼104.7 lx at the summer solstice with diurnal variability of 5 lx (Fig. 10e and f). This level of light intensity is far above the threshold for triggering DVM. At ±7 d from the summer solstice, there is no statistically significant correlation between MVBS and light intensity for the subsurface water layer at both Anabar and Khatanga (Fig. 10e and f), indicating no DVM occurs. Actograms in Figs. 4c–g and 5c–g do not show DVM during polar day either. Note, however, that the DVM signal in the Laptev Sea has already ceased advancing polar day (Figs. 4 and 5). A surface-intensified enhancement of MVBS at Anabar during the first part of June 2008 (Fig. 4c–g) seemed to be impacted by intrusions of turbid water generated by the Lena River runoff.
In the Beaufort Sea, light intensity below the ice is rather similar to that of the Laptev Sea (Figs. 4–7b). CA05 and CA08 became ice-free shortly after the summer solstice, lagging ice retreat in the Laptev Sea by about 1.5 months (Figs. 4–7a). Sea ice retreat at CA05 and CA08 results in a 30-fold increase in the surface layer irradiance up to the level simulated for the Laptev Sea (Figs. 6b and 7b). Around summer solstice at CA05, statistically significant correlation between MVBS and light intensity (0.34) indicates occurrence of DVM at the subsurface water layer (Fig. 10g), while it is not obvious from the actogram in Fig. 6c. In the deeper water layer, DVM below the ice is confirmed until summer solstice by the difference in MVBS from midnight to noon (Fig. 6d–g). However, this signal gradually vanishes at the beginning of July, when CA05 became free of ice (Fig. 6a). For the subsurface layer at CA08, there is no correlation between MVBS and light intensity around summer solstice (Fig. 10h). However, MVBS actograms for the deeper layer clearly show DVM during polar day until the ice started to retreat before the beginning of July 2006 (Fig. 7d–g).
Actograms for the ADCP-measured vertical velocity in the Beaufort Sea show that the DVM pattern is hardly recognizable after the beginning of May 2006 when the polar day began (Fig. 8). However, net downward and upward flow remains traceable before and after midnight, respectively, until the end of June 2006 (Fig. 8). Note that the range of vertical velocity 0.1 cm s−1 associated with this flow may be within the noise level of velocity measurements.
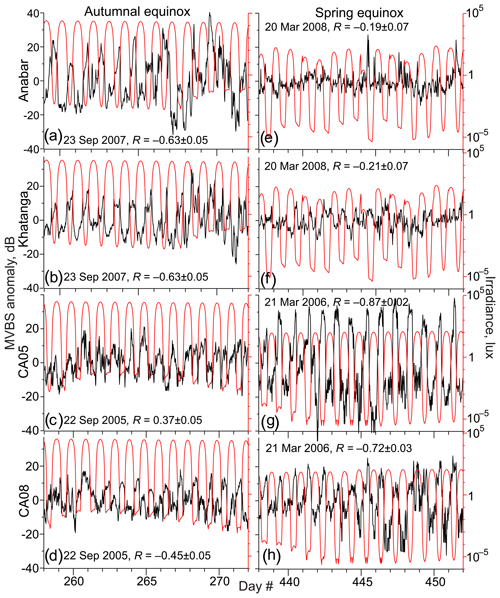
Figure 11Time series of MVBS anomaly (black, dB) relative to the 7 d running mean for the ADCP uppermost level and modeled under-ice irradiance (lx) in a decimal logarithm scale (red) for 7 d before and after the autumnal (left) and spring (right) equinox for (a, e) Anabar, (b, f) Khatanga, (c, g) CA05, and (d, h) CA08. Correlation R between the MVBS anomaly and under-ice irradiance estimated for the 95 % confidence level.
3.4 Diurnal signal of the mean volume backscatter strength during transitional periods
In the Laptev Sea, during the fall transition from polar day to polar night (approximately to the beginning of November 2007), the occurrence of the MVBS diurnal pattern corresponds to a decrease in the surface layer irradiance after sunset below 3.3 lx and 0.8 lx for Anabar and Khatanga, respectively (Fig. 9a and b). This irradiance threshold is associated with light intensity during the deep twilight (see Sect. 3.1 for more details). The MVBS diurnal signal roughly follows the irradiance threshold, with acoustic backscatter enhanced during the dark period. Negative correlation between MVBS and irradiance ± 7 d from the autumnal equinox at both Anabar and Khatanga (0.63; Fig. 11a and b) confirms DVM during transition from polar day to polar night. After the beginning of polar night, once the under-ice irradiance falls below irradiance threshold for 24 h a day, the MVBS diurnal pattern vanishes, lagging behind the beginning of polar night by about 2 weeks (Figs. 4b–g and 5b–g). This is a period when the twilight still persists after sunset. In the Laptev Sea, DVM during fall (and spring) was persistent down to 24 m at Anabar (Fig. 4c–f) and to 30 m at Khatanga (Fig. 5c–f).
In the Beaufort Sea, the transitional period from polar day to polar night in September to November 2005 is characterized by MVBS diurnal patterns similar to those in the Laptev Sea (Figs. 6 and 7). However, the light threshold for triggering DVM is about 2 times to 1 order of magnitude lower compared to the Laptev Sea (see Sect. 3.1). In the Beaufort Sea, seasonal DVM during transitions from polar day to polar night (and vice versa) was observed through the entire depth range of the ADCP profiling (Figs. 6c–g and 7c–g). At ± 7 d from the autumnal equinox, negative correlation between MVBS and irradiance clearly indicates DVM at CA08 (0.45; Fig. 11d). In contrast, positive correlation at CA05 (R=0.37; Fig. 11c) suggests reversing DVM through the subsurface water layer as also follows from the actogram in Fig. 6c. Moonlight and/or upwelling seem to cause the to DVM reverse at CA05 during this time. In contrast to the Laptev Sea, DVM in the Beaufort Sea was not disrupted following the beginning of polar night, as discussed in section 3.3 (Figs. 6, 7, 10c, and d).
The MVBS diurnal pattern resumed (Laptev Sea) and enhanced (Beaufort Sea) during spring transition from polar night to polar day. This corresponds to a daily increase in the under-ice irradiance to the level exceeding light threshold revealed in Sect. 3.1. Negative correlation between MVBS and irradiance at ± 7 d from the spring equinox for all four moorings (Fig. 11e–h) indicates DVM in the subsurface water layer. However, the relationship between irradiance and MVBS was disrupted approaching and following the beginning of polar day in the Laptev and Beaufort seas, respectively.
In the Laptev Sea, the DVM signal shallowed, vanishing at 24–27 and 35 m depth at Anabar and Khatanga, respectively (Figs. 4f, 4g, and 5g). For the overlying water layer, a daily cycle in MVBS was mimicked by the diurnal cycle in the sun irradiance until March 2007 (Figs. 4 and 5). From this point, the MVBS diurnal signal ceased lagging polar day by about 1 and 1.5 months for Khatanga and Anabar, respectively (Figs. 4 and 5). At Anabar, DVM disappeared in mid-March preceding full moon event no. 7 (Fig. 4). At Khatanga, DVM abruptly vanished on 26 March 2008, approximately 1 month prior to the start of polar day when the MVBS diurnal signal would be expected to end. In fact, at Anabar and Khatanga there was no difference in MVBS between midnight and noon, indicating disappearance of the diurnal rhythm already before the beginning of polar day. Note that from the mid-March 2007 the polynya started to develop in the Laptev Sea (Fig. 2), but both moorings were still located below the pack ice of ∼1.7 m thick (Figs. 4a and 5a).
In the Beaufort Sea, the MVBS diurnal signal extended relative to the beginning of polar day by about 1.5 months. At 15–16 m depth, diurnal signal followed the 0.3–0.4 lx threshold and vanished once the under-ice irradiance exceeded the light threshold throughout the day shortly before the start of polar day at the beginning of May 2006 (Figs. 6c and 7c). During this time, CA05 and CA08 were still located beneath the pack ice that was ∼2 m thick (Figs. 6a and 7a). In contrast to the subsurface water layer, MVBS diurnal pattern at deeper water layers maintained their identity during the first half of polar day and vanished shortly after the summer solstice (Figs. 6d–g and 7d–g). Here, DVM was disrupted only at the end of June 2007 once the sea ice started to deteriorate after 27 June 2006 (Figs. 3, 6, and 7).
4.1 On the light threshold for triggering DVM in the Laptev and Beaufort seas
Our acoustic backscatter analysis shows that the light threshold for triggering DVM in the Laptev Sea is about 2 to 10 times higher compared to the Beaufort Sea. However, this analysis is limited by lack of zooplankton observations. It is logistically difficult to sample zooplankton in the seasonally ice-covered and remote areas of the Arctic shelf; hence, it is unclear which zooplankton species were likely to undergo DVM. Acknowledging this deficiency, the difference in zooplankton response to irradiance in the Laptev and Beaufort seas could potentially be attributed to the three different factors described below.
First, different zooplankton species respond to specific wavelengths (Cohen et al., 2015; Cohen and Forward, 2002). It is possible that different zooplankton communities occurred in the Laptev and Beaufort seas, reacting differently to wavelengths at a specific time of the year. It is also possible that lower turbidity levels and CDOM concentrations in the Beaufort Sea resulted in different wavelengths in the Beaufort and Laptev seas.
Second, although some studies have not found any impact on the response of zooplankton to light in relation to temperature (e.g., Cohen and Frank, 2006), others have found that krill have higher light sensitivity at cold temperatures (Jonathan Cohen, University of Delaware, personal communication, 2020). Moreover, temperature can also induce changes in the irradiance sensitivity of some zooplankton, and a temperature-induced change in the animal's phototactic reactions may impact the DVM response to irradiance (Bandara et al., 2021). The lower surface temperatures prevailing in the Beaufort Sea (Figs. S3 and S5) could thus partly explain the difference in seasonal DVM patterns.
The third and most likely reason behind the different DVM response to irradiance is related to bottom depth (<50 m in the Laptev Sea and 200–400 m in the Beaufort Sea). In the Arctic Ocean, including its marginal seas, DVM are documented for several large-sized abundant Arctic copepods, such as Metridia longa and Calanus glacialis; smaller-sized Pseudocalanus spp.; mysids; and the chaethognath Parasagitta elegans (Runge and Ingram, 1991; Daase et al., 2008; Vestheim et al., 2013). Most of the listed species are inhabitants of relatively deep Arctic shelf regions with depths >50 m (Conover and Huntley, 1991; Kosobokova et al., 1998; Darnis et al., 2022; Skjoldal and Aarflot, 2023). In the shallower regions, like the sites where Anabar and Khatanga were deployed in the Laptev Sea (30–40 m), only copepods like Pseudocalanus spp. and mysids could be regarded as migrators, but they are numerous in these regions only during the ice-free summer period (Abramova, 1999). In the deeper Beaufort Sea, in contrast, all the listed species migrators are abundant during the entire year and could potentially perform DVM depending on irradiance cycle. Due to the pronounced differences in the composition of zooplankton communities in the shallow Laptev Sea and much deeper region of the Amundsen Gulf in the Beaufort Sea, one can expect quite different patterns of DVM in these two regions that we do actually observe using acoustic methods. Moreover, the very shallow Laptev Sea restrained the amplitude of the DVM, and it is possible that the difference in irradiance between the surface and seafloor was not strong enough to trigger DVM under low-light conditions. It is also possible that planktivorous fish such as polar cod migrate to deeper areas in winter, a period during which they prefer warmer and deeper Atlantic waters (Geoffroy et al., 2011), thus avoiding the location of the Laptev Sea moorings. The absence of visual predators during that period would thus hinder the need for DVM during the polar night in the Laptev Sea because DVM is due to a combination of grazing needs and predator avoidance.
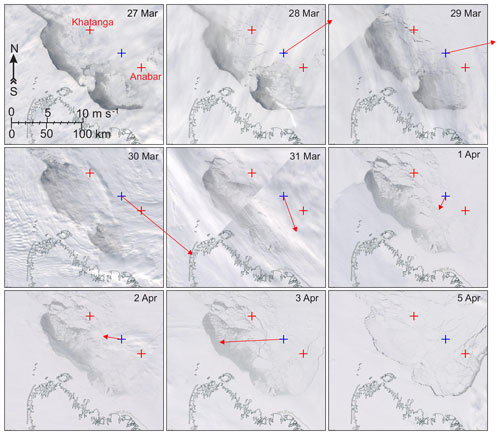
Figure 12The MODIS true-color satellite imagery for 27 March to 5 April 2008 showing the evolution of polynya in a relatively cloud-free area northward of the Lena Riven delta (Laptev Sea). Red crosses mark the moorings at Khatanga and Anabar. Blue crosses depict the spatial node where 2 m air temperature and 10 m wind were derived from ERA5 (Fig. S2a and b, respectively). Red arrows show daily mean wind for the day the image was taken. No arrows indicates no wind.
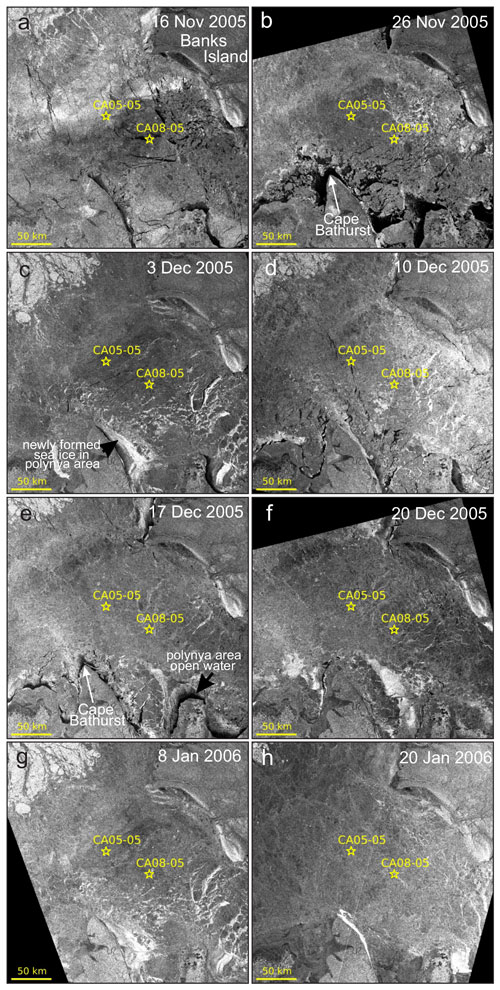
Figure 13RADARSAT-1 ScanSAR satellite images showing the evolution of ice conditions in the Amundsen Gulf in (a, b) November 2005, (c–f) December 2005, and (g, h) January 2006. Yellow stars depict moorings CA05 and CA08. The area with low radar backscatter corresponds to calm open water in leads and polynyas. The gray strips are associated with a thin sea ice that is newly formed in leads and polynyas.
4.2 Sea ice impact on DVM
Actograms in Figs. 4–8 show that DVM in the Laptev and Beaufort seas was in general controlled by light attenuated by the first-year pack ice. This pattern, however, was deviated from about 1.5 months before and after the beginning of polar day in the Laptev and Beaufort seas, respectively. We speculate that the disappearance of DVM in the Laptev Sea before the beginning of polar day is attributed to the polynya opening. In the Beaufort Sea, the DVM has been extended over the polar day to the summer solstice. We attribute this extension to light attenuation by the first-year pack ice. In this section, we examine these suggestions in more detail using sea ice satellite imagery (Figs. 12 and 13). Moreover, for elucidating the impact of sea ice disruptions on DVM in the Laptev and Beaufort seas, we use time series of correlations between subsurface MVBS and irradiance.
4.2.1 Polynyas and leads
By the end of polar night and until mid-May 2007, Anabar was located below the first-year pack ice of ∼1.7 m thick in the Laptev Sea. At Khatanga, the first-year pack ice started deteriorating before the end of June 2008 (Fig. 2). In mid-March, the southeasterly winds up to 8 m s−1 forced the development of the coastal polynya ∼15 km from Anabar (Figs. 2 and S2b). This corresponds to disappearance of DVM at Anabar (Fig. 4c–g). On 26 and 28–29 March 2008, two consecutive events of southwesterly winds generated polynya open water that extended ∼30 km off the landfast ice edge and for ∼15–30 km to Anabar and Khatanga, where the ice cover strongly deteriorated (Figs. 2 and 12). This is consistent with abrupt cessation of DVM at Khatanga (Fig. 5c–g), which was accompanied by the bottom-intensified increase in MVBS of ∼45 dB. Strong southeasterly winds during the second part of April 2008 (Fig. S2b) extended the polynya area in the southeastern Laptev Sea, and Anabar was located below the polynya open water up to the end of April (Figs. 2 and 4a). Thus, using the analysis of wind and satellite imagery we reveal that the DVM disruptions in the Laptev Sea are likely attributed to the polynya openings.
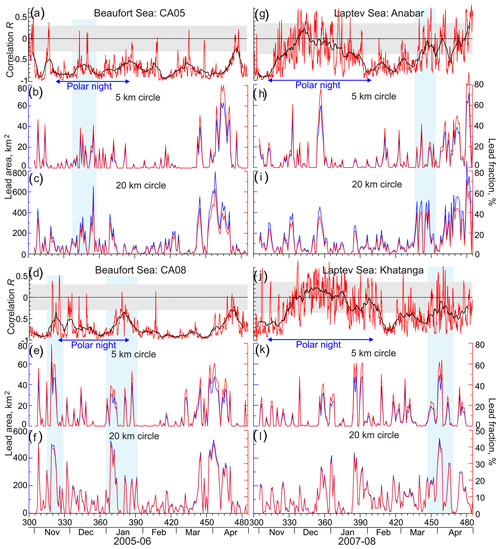
Figure 14Correlation R between the mean volume backscatter strength at the ADCP uppermost level and modeled irradiance computed for the 1 d moving window (red) with their 7 d running mean (black) for (a) CA05, (d) CA08, (g) Anabar, and (j) Khatanga. Gray shading highlights statistically insignificant correlations at the 99 % confidence level. Blue arrows at the bottom indicate the period of polar night. Blue shading highlights disruptions in DVM attributed to polynyas and leads (b, c, e, f, h, i, k, l). Time series of the lead area (blue, km2) and fraction (red, %) derived from the MODIS ArcLead dataset (Reiser et al., 2020) for the 5 km (b, h, e, k) and 20 km (c, i, f, l) radius circles centered at mooring positions.
We suggest that the deviations or disruptions in the correlation between subsurface MVBS and light can be the measure for polynya impact on DVM. In the Laptev Sea, during a major portion of polar night when there is no twilight to observe, there is no statistically significant correlation between MVBS and light (Fig. 14g and j), indicating no DVM. Transition from polar night to polar day is characterized by statistically significant correlation 0.35 revealing DVM. At Anabar, this relationship was disrupted in mid-March following several consecutive events of polynya opening nearby Anabar (Figs. 2, 12, 14h, and i). From the beginning of April 2008, correlation decreases consistently with increases in the lead fraction (i.e., open water and thin ice) derived from MODIS thermal infrared imagery (Fig. 14h and i). Finally, correlation falls to zero on ∼26 April (Fig. 14g) when Anabar became free of ice (Fig. 2). At Khatanga, correlation between MVBS and light was completely disrupted during the last decade of March 2008 following consecutive events of polynya opening 5–20 km from the station (Figs. 2, 12, 14k, and l). This is evidence for the polynya's impact on DVM as Khatanga became free of ice only in mid-July 2008 (Fig. 2). Similar to these results, Dmitrenko et al. (2021) reported that the formation of the Laptev Sea coastal polynya in late March 1999 caused DVM to abruptly cease near the landfast ice edge, while DVM persisted through spring to the start of polar day at the onshore mooring.
During the major portion of polar night in the Beaufort Sea, negative correlations between MVBS and irradiance were statistically significant (Fig. 14a and d), indicating DVM as discussed in Sect. 3.2. However, there were several short-term events when negative correlations become statistically insignificant. At CA05, disruption of DVM in December 2005 (Fig. 14a) is attributed to deterioration of ice pack and formation of leads in the vicinity of CA05 (Figs. 13c–f, 14b, and c) forced by northeasterly winds (Fig. S2d). At CA08, two lead events during the second part of November 2005 and in January 2006 (Figs. 13a, b, g, h, 14e, and f) deviated in correlation between MVBS and irradiance disputing the presence of DVM (Fig. 14d). The polynya opening on 17–20 March 2006 in the vicinity of CA05 (Figs. 3, 14b and c) did not disrupt DVM at 16 m depth. However, the deeper water layer shows deviations in DVM attributed to polynya opening (Fig. 6d–g). Note, however, that lead events from the end of March to mid-April did not cause a deviation in DVM at CA05 and CA08. During these events DVM was totally driven by light.
Our observations suggest that DVM is not solely controlled by daily changes in irradiance, as there were no abrupt changes in the under-ice light conditions while the Laptev Sea polynya was open during March–April 2008, and both Anabar and Khatanga were located at a sizable distance from polynya open water (Figs. 2, 3a, 4a, and 12). In the Beaufort Sea, the appearance of open water is mainly attributed to deterioration of the ice pack and formation of leads in the vicinity of CA05 and CA08 (Figs. 3 and 13). Only the open-water event on 17–20 March 2006 can be attributed to the polynya opening, but CA05 was located at a sizable distance from polynya open water (Fig. 3).
Following Dmitrenko et al. (2021), we suggest that formation of polynyas and leads generates far-field effects on zooplankton behavior. Since the opening of the polynya and leads did not cause any immediate change in irradiance at moorings, the observed deviations in DVM could potentially be explained by predator-avoidance behavior of zooplankton. Polar cod (Boreogadus saida) is one of the main predators of the zooplankton taxa likely responsible for the observed DVM (e.g., Buckley and Whitehouse, 2017; Cusa et al., 2019; Bouchard and Fortier, 2020; Aune et al., 2021; Geoffroy et al., 2023). Among other habitats, polar cod is known to occupy surface waters over shallow coastal continental shelves in winter and spring (e.g., Gradinger and Bluhm, 2004; David et al., 2016). Polynya and lead openings may increase zooplankton mortality by increasing the predation pressure from polar cod because more light from polynya openings increases the prey detection distance of polar cod, improving its capacity to forage on zooplankton (Bouchard and Fortier, 2008; Jönsson et al., 2014; Varpe et al., 2015; Langbehn and Varpe, 2017; Cusa et al., 2019). The zooplankton possibly stopped conducting DVM and remained closer to the seafloor as a predator-avoidance behavioral response to the increase in polar cod abundance in the photic zone near the surface.
In the Laptev Sea, DVM disruptions were observed in response to polynya openings during the entire transitional period from polar night to polar day (Figs. 2, 4, 5, and 14g–l). In the Beaufort Sea, DVM partially deviated in response to formation of leads in November to January. However, in contrast to the Laptev Sea, DVM in the Beaufort Sea did not deviate in response to several significant events of open water observed in March–April 2006 (Figs. 3 and 14a–f). Despite the open water in the vicinity of moorings, negative correlations between MVBS and irradiance remain statistically significant (e.g., Fig. 11g and h). We attribute this difference to the seasonal behavior of polar cod. Aggregations of polar cod in the Amundsen Gulf were observed from December to mid-March (Geoffroy et al., 2011). Dissipation of the polar cod aggregation to March–April eliminates the predators' risk, and zooplankton resumes DVM during polynya and lead events, as seen in Fig. 17a–f. This suggests that a low-predation-pressure environment in the Amundsen Gulf during spring allows zooplankton to conduct DVM during the formation of polynyas and leads. Another possible reason is seasonal migration of polar cod to deeper and warmer Atlantic waters (Geoffroy et al., 2011) located in the Amundsen Gulf below 220–250 m depth (Figs. S3d, S5a, and d).
4.2.2 First-year pack ice
Our data from the Beaufort Sea show that DVM ceased only at 15 m and 16 m of depth at CA08 and CA05, respectively (Figs. 5c and 6c), during polar day in 2006. In the underlying water layer, DVM continued until the beginning of July 2006 (Figs. 5d–g and 6d–g). It seems that zooplankton were conducting DVM, but they were still avoiding relatively well-illuminated subsurface water. This is in line with predator-avoidance behavior during transitional seasons but without seasonal modulation because the sun is above the horizon 24 h a day. We speculate that the light intensity at depths exceeding 15–16 m was below the threshold of predator perception, allowing DVM at these depths to extend over polar day to the summer solstice in 2006.
We suggest that the polar day DVM in 2006 was likely generated by attenuation of light caused by the first-year pack ice that was ∼1.8 m thick. During the first part of polar day during the summer solstice, the subsurface under-ice water layer in the Beaufort Sea was illuminated to 103 lx, which is ∼100 times lower than in the Laptev Sea (Fig. 10e–h). This difference is entirely explained by light attenuation from the ice pack that persisted at CA05 and CA08 until the very beginning of July and retreated onward shortly after the summer solstice. Thus, our results from the Beaufort Sea highlight the important role of sea ice in promoting DVM during polar day when sunlight illuminates the surface water layer 24 h a day. Note that for the Beaufort Sea continental slope, Dmitrenko et al. (2020) reported DVM prolongation toward polar day due to the multiyear Greenland pack ice shading the under-ice water layer.
4.3 DVM disruptions related to wind-forced water dynamics and moonlight
Our results revealed that water dynamics temporally impact DVM by disrupting the diurnal rhythm. In the Laptev Sea, two wind-driven storms at the end of September and beginning of October 2007 with northerly winds exceeding 20 m s−1 resulted in vertical mixing of the water column down to the seafloor at Anabar (Hölemann et al., 2011). These storm events completely modified initial vertical distributions of temperature and salinity shown in Fig. S3a, generating positive downward heat flux and negative salt flux (Hölemann et al., 2011). The actograms of MVBS at Anabar show that DVM was disrupted down to the seafloor following these two storms (Fig. 3c–g). At Khatanga, MVBS signal was becoming noisier, but DVM remained traceable (Fig. 4c–g).
In the Beaufort Sea, three consecutive upwelling-favorable storms with northeasterly winds exceeding 10 m s−1 were observed from the end of September to the end of October 2007 before freeze-up onset (Fig. S2d). Upwelling of nutrient-rich, Pacific-origin water to the surface at Cape Bathurst was commonly observed (Williams and Carmack, 2008). For example, upwelling of cold and saline water on the western flank of the Amundsen Gulf was observed in October 2006 (Fig. S5d and e), about 2.5 months after CA05 and CA08 were recovered in July 2006. In winter, the upwelling-favorable wind forces the mobile pack ice to move off the stationary landfast ice edge forming the Cape Bathurst polynya.
The actograms of vertical velocity from CA08 show deviations in DVM consistent with three upwelling-favorable storms in September–October 2005 (Figs. 8b and S2d). A net upward flux typical of coastal upwelling seems to be superimposed on DVM, partially masking the DVM signal. At CA05, the deviations imposed by coastal upwelling were less recognizable (Fig. 8a). Actograms of MVBS for CA05 and CA08, however, do not show DVM disruptions that can be attributed to the upwelling-favorable storms.
Wind forcing also plays a role in the formation of enhanced acoustic scattering on the top of MVBS signal generated by DVM. In the Laptev Sea, we observed high MVBS events that can be attributed to the frazil ice crystals and riverine suspended sediments (e.g., Wegner et al., 2005; Dmitrenko et al., 2010a; Ito et al., 2020). An enhanced acoustic backscatter was recorded following the wind-driven open-water events at Anabar during the very end of April and mid-May 2008 when sea ice concentration fell below 90 % (Figs. 4a, c, and S2a). The frazil ice was likely generated by new ice growth within the polynya open water during these two periods when air temperatures remained relatively cold (−12 and −7 °C in April and May 2008, respectively; Fig. S2a).
In contrast to MVBS events in April–May, the enhanced acoustic scattering in July–August at Anabar and Khatanga (Figs. 4c and 5c) seems to be attributed to intrusions of turbid water associated with river runoff. The Lena River plume is enriched with suspended sediments (e.g., Pivovarov et al., 1999; Wegner et al., 2005). We suggest that intrusions of turbid riverine water generated high MVBS at Anabar and Khatanga in July–August 2008. During July 2008, northeasterly winds (Fig. S1e) resulted in divergence of the Lena River plume into the southeastern Laptev Sea, the area where Anabar and Khatanga were located (Figs. S3a, b, and S4b; for more details see Dmitrenko et al., 2010), generating enhanced MVBS at the front of the Lena River delta. For the southeastern Beaufort Sea, the southwesterly winds that dominated during summer 2006 (Fig. S1b and c) are suggested to force eastward transport of the Mackenzie River water away from the Amundsen Gulf where CA05 and CA08 were deployed. Hence, the signature of riverine-water-suspended sediments is not traceable in MVBS actograms during summer 2006 (Figs. 6 and 7).
Finally, MVBS actograms in Figs. 4–7 suggest no deviation of the MVBS diurnal pattern that can be associated with moonlight. During full moons, the midnight under-ice irradiance increased up to 0.01 lx in October and 0.001 lx in February–March (Figs. 4–7a). This level of light intensity is far below the threshold revealed in Sect. 5.1. Furthermore, the uncertain data regarding the total cloud cover from the Aqua/MODIS revealed high cloudiness until November when the sea ice thickness did not exceed 50 cm (Figs. 4–7a) that make any suggestions about the impact of moonlight on DVM before the beginning of polar night very speculative. Afterwards, during polar night the mean cloudiness decreases to 5 %–10 %, but gradually growing sea ice thickness and snow depth both increase light attenuation limiting under-ice light intensity to 0.001 lx. However, the moonlight impact on DVM cannot be completely neglected. The DVM reversal at CA05 in the end of September 2005 was interpreted as a result of upwelling due to favorable wind forcing (Figs. 6c and S2d). Alternatively, it can be explained by the moonlight during the full moon event no. 1 when the midnight light intensity was slightly below 0.1 lx (Fig. 11c). Note that during this period the total cloud cover varied from 20 % to 100 % (Fig. 6b).
Based on yearlong mooring observations from the southeastern shelves of the Laptev and Beaufort seas, both of which are in the vicinity of the circumpolar Arctic coastal polynya, we conclude that DVM in both areas is influenced by the formation of coastal polynyas and leads and the associated increase in light transmittance, despite the light threshold for triggering DVM in the Laptev Sea being about 2 times to 1 order of magnitude higher than what is observed in the Beaufort Sea. Along with a deeper and more southerly location of the mooring array in the Beaufort Sea, a lower light threshold allows DVM to occur in the Beaufort Sea during polar night, whereas DVM ceases in the Laptev Sea. We attribute this different pattern in DVM to different zooplankton communities, different wavelengths and water temperatures, and/or a reduced predation pressure due to the shallower waters of the Laptev Sea.
In the Laptev Sea, during the spring transition from polar night to polar day, DVM ceased ∼1 month prior to its expected cessation at the start of polar day. We associate this disruption with predator-avoidance behavior of zooplankton conditioned by higher polar cod abundance attracted by the polynya opening. In the Beaufort Sea, leads cause DVM to deviate only during winter when aggregations of polar cod are observed. During spring, a low-predation-pressure environment favors zooplankton conducting DVM during polynya and lead events. In the Laptev Sea, the acoustic backscatter during polar day, when DVM has stopped, is caused by scattering from suspended sediments within the Lena River plume. In the Beaufort Sea, the first-year pack ice prolonged the occurrence of DVM toward the polar day due to the sea ice shading the under-ice water layer.
Overall, our results highlight the role of sea ice in disrupting synchronized DVM in the vicinity of polynyas and leads and in promoting DVM below the ice during polar day. In general, increasing light penetration through polynyas and leads reduces the under-ice habitat of zooplankton. In contrast, an extension of the ice-covered period favors the under-ice habitat of zooplankton. This is in agreement with the conclusions of Flores et al. (2023), who suggested the deepening of zooplankton in response to sea ice decline. Given the fact that the size of the Laptev Sea and Cape Bathurst polynyas during winter has tended to increase over the last 2 decades (Preußer et al., 2019), the cessation of DVM in the surrounding areas could be important for the ecology of Arctic zooplankton and may disrupt the biological pump, which has implications for carbon fluxes (e.g., Kelly et al., 2019; Archibald et al., 2019).
The ADCP data that support the findings of this study are openly available from Mendeley Data at https://doi.org/10.17632/75rk5kbnn4.1 (Dmitrenko, 2024). They are in a binary instrumental format and can be implemented as assets using WinADCP, a software package for use with RDI acoustic Doppler current profilers for the interactive exploration, analysis, and visualization of ADCP data. WinADCP documentation can be found at https://www.comm-tec.com/prods/mfgs/RDI/Software/Manuals/WinADCP-Manual/WinADCP User Guide.pdf (Teledyne RD Instruments, 2001).
The supplement related to this article is available online at: https://doi.org/10.5194/os-20-1677-2024-supplement.
IAD and VP guided the overall research problem and developed the methodology. IAD, MG, and KK conceptualized this research. IAD, VP, ASK, AP, and SAK conducted the formal analyses and data curation. SAK performed the field research. DBar allocated resources. IAD, KK, AP, and MG wrote the original draft. IAD, AP, KK, ASK, MG, CB, and DBab revised and edited the original draft. IAD, VP, and AK generated the figures. IAD and DBar supervised and administrated the project. DBar organized the funding acquisition. All authors contributed to the article and approved of the submitted version.
The contact author has declared that none of the authors has any competing interests.
Publisher's note: Copernicus Publications remains neutral with regard to jurisdictional claims made in the text, published maps, institutional affiliations, or any other geographical representation in this paper. While Copernicus Publications makes every effort to include appropriate place names, the final responsibility lies with the authors.
We dedicate this paper to our colleague David Barber (1960–2022). Through his vision, leadership, and endless efforts, David Barber was a visionary researcher with a passion for the Arctic, a scholar with an entrepreneurial spirit, and a generous mentor and friend. We thank NASA and the Alaska Satellite Facility for granting access to RADARSAT-1 imagery used in this study.
Field research in the Laptev Sea was funded through the Bundesministerium für Bildung und Forschung projects “System Laptev Sea”. Field research in the Beaufort Sea was conducted as part of the Canadian ArcticNet field program. Funding for this work was provided by the Canada Excellence Research Chairs (CERC) program led by Dorthe Dahl-Jensen. The work of Ksenia Kosobokova was funded by the Russian Science Foundation (grant no. 23-17-00121) and carried out within the framework of the state assignment of the IORAS (theme no. FMWE-2024-0021). Andreas Preußer was supported by the European Union's Horizon 2020 Research and Innovation Programme (grant no. 101003472) and the German Federal Ministry of Education and Research (Bundesministerium für Bildung und Forschung – BMBF; grant no. 03F0831C (CATS-Synthesis)).
This paper was edited by Agnieszka Beszczynska-Möller and reviewed by one anonymous referee.
Abramova, E.: Composition, abundance and population structure of spring-time zooplankton in the shelf-zone of the Laptev Sea, in: Land-Ocean Systems in the Siberian Arctic: Dynamics and History, edited by: Kassens, H., Bauch, H. A., Dmitrenko, I. A., Eicken, H., Hubberten, H.-W., Melles, M., Thiede, J., and Timokhov, L. A., Springer-Verlag, Berlin, Germany, 161–168, https://doi.org/10.1007/978-3-642-60134-7_16, 1999.
Abramova, E. and Tuschling, K.: A 12-year study of the seasonal and interannual dynamics of mesozooplankton in the Laptev Sea: Significance of salinity regime and life cycle patterns, Global Planet. Change, 48, 141–164, https://doi.org/10.1016/j.gloplacha.2004.12.010, 2005.
Ackerman, S., Frey, R., Strabala, K., Liu, Y., Gumley, L., Baum, B., and Menzel, P.: Discriminating Clear-Sky from Cloud with MODIS Algorithm Theoretical Basis Document (MOD35), Version 6.1. MODIS Cloud Mask Team, Cooperative Institute for Meteorological Satellite Studies, University of Wisconsin: Madison, WI, USA, https://modis.gsfc.nasa.gov/data/atbd/atbd_mod06.pdf (last access: 22 October 2024), 2010.
Arashkevich, E. G., Drits, A. V., Pasternak, A. F., Flint, M. V., Demidov, A. B., Amelina, A. B., Kravchishina, M. D., Sukhanova, I. N., and Shchuka, S. A.: Distribution and Feeding of Herbivorous Zooplankton in the Laptev Sea, Oceanology, 58, 381–395, https://doi.org/10.1134/S0001437018030013, 2018.
Archibald, K., Siegel, D. A., and Doney, S. C.: Modeling the impact of zooplankton diel vertical migration on the carbon export flux of the biological pump, Global Biogeochem. Cy., 33, 181–199, https://doi.org/10.1029/2018GB005983, 2019.
Arrigo, K. R.: Chapter 7 Physical Control of Primary Productivity in Arctic and Antarctic Polynyas, in: Polynyas: Windows to the World, edited by: Smith, W. O. and Barber D. G., Elsevier Oceanography Series, 74, 223–238, https://doi.org/10.1016/S0422-9894(06)74007-7, 2007.
Arrigo, K. R. and van Dijken, G. L.: Annual cycles of sea ice and phytoplankton in Cape Bathurst polynya, southeastern Beaufort Sea, Canadian Arctic, Geophys. Res. Lett., 31, L08304, https://doi.org/10.1029/2003GL018978, 2004.
Aune, M., Raskhozheva, E., Andrade, H., Augustine, S., Bambulyak, A., Camus, L., Carroll, J. L., Dolgov, A. V., Hop, H., Moiseev, D., Renaud, P. E., and Varpe, Ø.: Distribution and ecology of polar cod (Boreogadus saida) in the eastern Barents Sea: a review of historical literature, Mar. Environ. Res., 166, 105262, https://doi.org/10.1016/j.marenvres.2021.105262, 2021.
Bandara, K., Varpe, Ø., Wijewardene, L., Tverberg, V., and Eiane, K.: Two hundred years of zooplankton vertical migration research, Biol. Rev., 96, 1077–1693, https://doi.org/10.1111/brv.12715, 2021.
Barber, D. G. and Hanesiak, J. M.: Meteorological forcing of sea ice concentrations in the southern Beaufort Sea over the period 1979 to 2000, J. Geophys. Res.-Oceans, 109, C06014, https://doi.org/10.1029/2003JC002027, 2004.
Barber, D. G. and Massom, R.: Chapter 1 The Role of Sea Ice in Arctic and Antarctic Polynyas, in: Polynyas: Windows to the World, edited by: Smith, W. O. and Barber D. G., Elsevier Oceanography Series, 74, 1–54, https://doi.org/10.1016/S0422-9894(06)74001-6, 2007.
Barber, D. G., Asplin, M. G., Gratton, Y., Lukovich, J. V., Galley, R. J., Raddatz, R. L., and Leitch, D.: The International polar year (IPY) circumpolar flaw lead (CFL) system study: Overview and the physical system, Atmos.-Ocean, 48, 225–243, https://doi.org/10.3137/OC317.2010, 2010.
Belter, H. J., Janout, M., Hölemann, J. A., and Krumpen, T.: Data from: Daily Mean Sea Ice Draft From Moored Upward-Looking Acoustic Doppler Current Profilers (ADCPs) in the Laptev Sea from 2003 to 2016, PANGAEA [data set], https://doi.org/10.1594/PANGAEA.912927, 2020.
Belter, H. J., Krumpen, T., Janout, M. A., Ross, E., and Haas, C.: An Adaptive Approach to Derive Sea Ice Draft from Upward-Looking Acoustic Doppler Current Profilers (ADCPs), Validated by Upward-Looking Sonar (ULS) Data, Remote Sens., 13, 4335, https://doi.org/10.3390/rs13214335, 2021.
Benoit, D., Simard, Y., Gagné, J., Geoffroy, M., and Fortier, L.: From polar night to midnight sun: photoperiod, seal predation, and the diel vertical migrations of polar cod (Boreogadus saida) under landfast ice in the Arctic Ocean, Polar Biol., 33, 1505–1520, https://doi.org/10.1007/s00300-010-0840-x, 2010.
Berge, J., Cottier, F., Last, K. S., Varpe, Ø, Leu, E., Søreide, J., Eiane, K., Falk-Petersen, S., Willis, K., Nygård, H., Vogedes, D., Griffiths, C., Johnsen, G., Lorentzen, D., and Brierley, A. S.: Diel vertical migration of Arctic the zooplankton during the polar night, Biol. Lett., 5, 69–72, https://doi.org/10.1098/rsbl.2008.0484, 2009.
Berge, J., Renaud, P. E., Darnis, G., Cottier, F., Last, K., Gabrielsen, T. M., Johnsen, G., Seuthe, L., Weslawski, J. M., Leu, E., Moline, M., Nahrgang, J., Søreide, J. E., Varpe, Ø., Lønne, O. J., Daase, M., and Falk-Petersen, S.: In the dark: a review of ecosystem processes during the Arctic polar night, Prog. Oceanogr., 139, 258–271, https://doi.org/10.1016/j.pocean.2015.08.005, 2015.
Bianchi, D., Galbraith, E. D., Carozza, D. A., Mislan, K. A. S., and Stock, C. A.: Intensification of open-ocean oxygen depletion by vertically migrating animals, Nat. Geosci., 6, 545–548, https://doi.org/10.1038/ngeo1837, 2013.
Bouchard, C. and Fortier, L.: Effects of polynyas on the hatching season, early growth and survival of polar cod Boreogadus saida in the Laptev Sea, Mar. Ecol. Prog. Ser., 355, 247–256, https://doi.org/10.3354/meps07335, 2008.
Bouchard, C. and Fortier, L.: The importance of Calanus glacialis for the feeding success of young polar cod: a circumpolar synthesis, Polar Biol., 43, 1095–1107, https://doi.org/10.1007/s00300-020-02643-0, 2020.
Brierley, A. S.: Diel vertical migration, Curr. Biol., 24, R1074–R1076, https://doi.org/10.1016/j.cub.2014.08.054, 2014.
Buckley, T. and Whitehouse, A.: Variation in the diet of Arctic Cod (Boreogadus saida) in the Pacific Arctic and Bering Sea, Environ. Biol. Fish., 100, 421–442, https://doi.org/10.1007/s10641-016-0562-1, 2017.
Carmack, E. C. and Macdonald, R. W.: Oceanography of the Canadian Shelf of the Beaufort Sea: A setting for Marine Life, Arctic, 56, 29–45, https://doi.org/10.14430/arctic733, 2002.
Cavalieri, D. J., Parkinson, C. L., Gloersen, P., and Zwally, H. J.: Sea Ice Concentrations from Nimbus-7 SMMR and DMSP SSM/I-SSMIS Passive Microwave Data, Version 1, Boulder, Colorado, USA, NASA National Snow and Ice Data Center [data set], https://doi.org/10.5067/8GQ8LZQVL0VL, 1996.
Cavalieri, D. J., Markus, T., and Comiso, J. C.: AMSR-E/Aqua Daily L3 12.5 km Brightness Temperature, Sea Ice Concentration, & Snow Depth Polar Grids, Version 3, Boulder, Colorado, USA, NASA National Snow and Ice Data Center [data set], https://doi.org/10.5067/AMSR-E/AE_SI12.003, 2014.
Cohen, J. H. and Forward Jr., R. B.: Spectral sensitivity of vertically migrating marine copepods, Biol. Bull., 203, 307–314, https://doi.org/10.2307/1543573, 2002.
Cohen, J. H. and Frank, T. M.: Visual physiology of the Antarctic amphipod Abyssorchomene plebs, Biol. Bull., 211, 140–148, https://doi.org/10.2307/4134588, 2006.
Cohen, J. H., Berge, J., Moline, M. A., Sørensen, A.J., Last, K., Falk-Petersen, S., Renaud, P. E., Leu, E. S., Grenvald, J., Cottier, F., Cronin, H., Menze, S., Norgren, P., Varpe, Ø., Daase, M., Darnis, G., and Johnsen, G.: Is ambient light during the high Arctic polar night sufficient to act as a visual cue for zooplankton?, PLoS One, 10, e0126247, https://doi.org/10.1371/journal.pone.0126247, 2015.
Comiso, J. C., Cavalieri, D. J., Parkinson, C. L., and Gloersen, P.: Passive microwave algorithms for sea ice concentration: a comparison of two techniques, Remote Sens. Environ., 60, 357–384, https://doi.org/10.1016/S0034-4257(96)00220-9, 1997.
Conover, R. J. and Huntley, M.: Copepods in ice-covered seas – distribution, adaptations to seasonally limited food, metabolism, growth patterns and life cycle strategies in polar seas, J. Mar. Res., 2, 1–41, https://doi.org/10.1016/0924-7963(91)90011-I, 1991.
Conover, R. J. and Siferd, T. D.: Dark-Season Survival Strategies of Coastal Zone Zooplankton in the Canadian Arctic, Arctic, 46, 303–311, https://doi.org/10.14430/arctic1357, 1993.
Cottier, F. R., Tarling, G. A., Wold, A., and Falk-Petersen, S.: Unsynchronised and synchronised vertical migration of the zooplankton in a high Arctic fjord, Limnol. Oceanogr., 51, 2586–2599, https://doi.org/10.4319/lo.2006.51.6.2586, 2006.
Cusa, M., Berge, J., and Varpe, Ø.: Seasonal shifts in feeding patterns: individual and population realized specialization in a high Arctic fish, Ecol. Evol., 9, 11112–11121, https://doi.org/10.1002/ece3.5615, 2019.
Daase, M., Eiane, K., Aksnes, D. L., and Vogedes, D.: Vertical distribution of Calanus spp. and Metridia longa at four Arctic locations, Mar. Biol. Res., 4, 193–207, https://doi.org/10.1080/17451000801907948, 2008.
Darnis, G. and Fortier, L.: Zooplankton respiration and the export of carbon at depth in the Amundsen Gulf (Arctic Ocean), J. Geophys. Res.-Oceans, 117, C04013, https://doi.org/10.1029/2011JC007374, 2012.
Darnis, G. and Fortier, L.: Temperature, food and the seasonal vertical migration of key arctic copepods in the thermally stratified Amundsen Gulf (Beaufort Sea, Arctic Ocean), J. Plankton Res., 36, 1092–1108, https://doi.org/10.1093/plankt/fbu035, 2014.
Darnis, G., Geoffroy, M., Dezutter, T., Aubry, C., Massicotte, P., Brown, T., Babin, M., Cote, D., and Fortier, L.: Zooplankton assemblages along the North American Arctic: Ecological connectivity shaped by ocean circulation and bathymetry from the Chukchi Sea to Labrador Sea, Elementa. Sci. Anth., 10, 00053, https://doi.org/10.1525/elementa.2022.00053, 2022.
David, C., Lange, B., Krumpen, T., Schaafsma, F., van Franeker, J. A., and Flores, H.: Under-ice distribution of polar cod Boreogadus saida in the central Arctic Ocean and their association with sea-ice habitat properties, Polar Biol., 39, 981–994, https://doi.org/10.1007/s00300-015-1774-0, 2016.
Deines, K. L.: Backscatter estimation using broadband acoustic Doppler current profilers, in: Proceedings of the IEEE 6th Working Conference on Current Measurement Cat. No. 99CH36331, IEEE, 13 March 1999, San Diego, CA, USA, 249–253, https://doi.org/10.1109/CCM.1999.755249, 1999.
Dmitrenko, I.: ADCP data from moorings Khatanga, Anabar, CA05, and CA08, Mendeley Data, V1 [data set], https://doi.org/10.17632/75rk5kbnn4.1, 2024.
Dmitrenko, I., Kirillov, S., Eicken, H., and Markova, N.: Wind-driven summer surface hydrography of the eastern Siberian shelf, Geophys. Res. Lett., 32, L14613, https://doi.org/10.1029/2005GL023022, 2005a.
Dmitrenko, I., Tyshko, K., Kirillov, S., Hölemann, J., Eicken, H., and Kassens, H.: Impact of flaw polynyas on the hydrography of the Laptev Sea, Global Planet. Change, 48, 9–27, https://doi.org/10.1016/j.gloplacha.2004.12.016, 2005b.
Dmitrenko, I. A., Kirillov, S. A., and Tremblay, L.B.: The long-term and interannual variability of summer fresh water storage over the eastern Siberian shelf: Implication for climatic change, J. Geophys. Res.-Oceans, 113, C03007, https://doi.org/10.1029/2007JC004304, 2008.
Dmitrenko, I. A., Kirillov, S. A., Tremblay, L. B., Bauch, D., and Willmes, S.: Sea-ice production over the Laptev Sea shelf inferred from historical summer-to-winter hydrographic observations of 1960s–1990s, Geophys. Res. Lett., 36, L13605, https://doi.org/10.1029/2009GL038775, 2009.
Dmitrenko, I. A., Wegner, C., Kassens, H., Kirillov, S. A, Krumpen, T., Heinemann, G., Helbig, A., Schröder, D., Hölemann, J. A., Klagge, T., Tyshko, K. P., and Busche, T.: Observations of supercooling and frazil ice formation in the Laptev Sea coastal polynya, J. Geophys. Res.-Oceans, 115, C05015, https://doi.org/10.1029/2009JC005798, 2010a.
Dmitrenko, I. A., Kirillov, S. A., Krumpen, T., Makhotin, M., Abrahamsen, E. P., Willmes, S., Bloshkina, E., Hölemann, J. A., Kassens, H., and Wegner, C.: Wind-driven diversion of summer river runoff preconditions the Laptev Sea coastal polynya hydrography: Evidence from summer-to-winter hydrographic records of 2007–2009, Cont. Shelf Res., 30, 1656–1664, https://doi.org/10.1016/j.csr.2010.06.012, 2010b.
Dmitrenko, I. A., Kirillov, S. A., Bloshkina, E., and Lenn, Y.-D.: Tide-induced vertical mixing in the Laptev Sea coastal polynya, J. Geophys. Res.-Oceans, 117, C00G14, https://doi.org/10.1029/2011JC006966, 2012.
Dmitrenko, I. A., Kirillov, S. A., Forest, A., Gratton, Y., Volkov, D. L., Williams, W. J., Lukovich, J. V., Bélanger, C., and Barber, D. G.: Shelfbreak current over the Canadian Beaufort Sea continental slope: wind-driven events in January 2005, J. Geophys. Res.-Oceans, 121, 2447–2468, https://doi.org/10.1002/2015JC011514, 2016.
Dmitrenko, I. A., Kirillov, S. A., Myers, P. G., Forest, A., Tremblay, B., Lukovich, J. V., Gratton, Y., Rysgaard, S., and Barber, D. G.: Wind-forced depth-dependent currents over the eastern Beaufort Sea continental slope: implications for pacific water transport, Elem. Sci. Anth., 6, 66, https://doi.org/10.1525/elementa.321, 2018.
Dmitrenko, I. A., Petrusevich, V., Darnis, G., Kirillov, S. A., Komarov, A. S., Ehn, J. K., Forest, A., Fortier, L., Rysgaard, S., and Barber, D. G.: Sea-ice and water dynamics and moonlight impact the acoustic backscatter diurnal signal over the eastern Beaufort Sea continental slope, Ocean Sci., 16, 1261–1283, https://doi.org/10.5194/os-16-1261-2020, 2020.
Dmitrenko, I. A., Petrusevich, V. Y., Kosobokova, K., Komarov, A. S., Bouchard, C., Geoffroy, M., Koldunov, N. V., Babb, D. G., Kirillov, S. A., and Barber, D. G.: Coastal Polynya Disrupts the Acoustic Backscatter Diurnal Signal Over the Eastern Laptev Sea Shelf, Front. Mar. Sci., 8, 791096, https://doi.org/10.3389/fmars.2021.791096, 2021.
Elias, S. A.: Overview: Ice Sheets and Polar Deserts – Ice of Life, Encyclopedia of the World's Biomes, 274–285, https://doi.org/10.1016/B978-0-12-409548-9.12118-9, 2020.
Flores, H., Veyssière, G., Castellani, G., Wilkinson, J., Hoppmann, M., Karcher, M., Valcic, L., Cornils, A., Geoffroy, M., Nicolaus, M., Niehoff, B., Priou, P., Schmidt, K., and Stroeve, J.: Sea-ice decline could keep zooplankton deeper for longer, Nat. Clim. Change, 13, 1122–1130, https://doi.org/10.1038/s41558-023-01779-1, 2023.
Gaston, K. J., Duffy, J. P., Gaston, S., Bennie, J., and Davies, T. W.: Human alteration of natural light cycles: causes and ecological consequences, Oecologia, 176, 917–931, https://doi.org/10.1007/s00442-014-3088-2, 2014.
Geoffroy, M., Robert, D., Darnis, G., and Fortier, L.: The aggregation of polar cod (Boreogadus saida) in the deep Atlantic layer of ice-covered Amundsen Gulf (Beaufort Sea) in winter, Polar Biol., 34, 1959–1971, https://doi.org/10.1007/s00300-011-1019-9, 2011.
Geoffroy, M., Majewski, A., LeBlanc, M., Gauthier, S., Walkusz, W., Reist, J. D., and Fortier, L.: Vertical segregation of age-0 and age-1+ polar cod (Boreogadus saida) over the annual cycle in the Canadian Beaufort Sea, Polar Biol., 39, 1023–1037, https://doi.org/10.1007/s00300-015-1811-z, 2016.
Geoffroy, M., Bouchard, C., Flores, H., Robert, D., Gjøsæter, H., Hoover, C., Hop, H., Hussey, N. E., Nahrgang, J., Steiner, N., Bender, M., Berge, J., Castellani, G., Chernova, N., Copeman, L., David, C. L., Deary, A., Divoky, G., Dolgov, A. V., Duffy-Anderson, J., Dupont, N., Durant, J. M., Elliott, K., Gauthier, S., Goldstein, E. D., Gradinger, R., Hedges, K., Herbig, J., Laurel, B., Loseto, L., Maes, S., Mark, F. C., Mosbech, A., Pedro, S., Pettitt-Wade, H., Prokopchuk, I., Renaud, P. E., Schembri, S., Vestfals, C., and Walkusz, W.: The circumpolar impacts of climate change and anthropogenic stressors on Arctic cod (Boreogadus saida) and its ecosystem, Elem. Sci. Anth., 11, 00097, https://doi.org/10.1525/elementa.2022.00097, 2023.
Gradinger, R. R. and Bluhm, B. A.: In-situ observations on the distribution and behavior of amphipods and Arctic cod (Boreogadus saida) under the sea ice of the high Arctic Canada Basin, Polar Biol., 27, 595–603, https://doi.org/10.1007/s00300-004-0630-4, 2004.
Gratton, Y., Guillot, P., Lago, V., Rail M.-E., and Simard, A.: ArcticNet 0501c – Beaufort Sea CTD data, Polar Data Catalogue, CCIN Ref. 449, 2014a.
Gratton, Y., Dumont, D., Rail, M.-E., Simard, A., and Thanassekos, S.: Data from: ArcticNet 0603a – Beaufort Sea CTD data, Polar Data Catalogue, CCIN Ref. 502, 2014b.
Grenfell, C. G. and Maykut, G. A.: The optical properties of ice and snow in the Arctic Basin, J. Glaciol., 18, 445–463, https://doi.org/10.3189/S0022143000021122, 1977.
Gutjahr, O., Heinemann, G., Preußer, A., Willmes, S., and Drüe, C.: Quantification of ice production in Laptev Sea polynyas and its sensitivity to thin-ice parameterizations in a regional climate model, The Cryosphere, 10, 2999–3019, https://doi.org/10.5194/tc-10-2999-2016, 2016.
Hall, D. K., Key, J. R., Casey, K. A., Riggs, G. A., and Cavalieri, D. J.: Sea-ice surface temperature product from MODIS, IEEE T. Geosci. Remote, 42, 1076–1087, https://doi.org/10.1109/TGRS.2004.825587, 2004.
Hays, G. C.: A review of the adaptive significance and ecosystem consequences of the zooplankton diel vertical migrations, Hydrobiologia, 503, 163–170, https://doi.org/10.1023/B:HYDR.0000008476.23617.b0, 2003.
Heide-Jørgensen, M. P., Burt, L. M., Hansen, R. G., Nielsen, N. H., Rasmussen, M., Fossette, S., and Stern, H.: The Significance of the North Water Polynya to Arctic Top Predators, Ambio, 42, 596–610, https://doi.org/10.1007/s13280-012-0357-3, 2013.
Hendricks, S., Paul, S., and Rinne, E.: ESA Sea Ice Climate Change Initiative (Sea_Ice_cci): Northern Hemisphere Sea Ice Thickness from the CryoSat-2 Satellite on a Monthly Grid (L3C), v2.0., Centre for Environmental Data Analysis [data set], https://doi.org/10.5285/ff79d140824f42dd92b204b4f1e9e7c2, 2018.
Hersbach, H., Bell, B., Berrisford, P., Hirahara, S., Horányi, A., Muñoz-Sabater, J., Nicolas, J., Peubey, C., Radu, R., Schepers, D., Simmons, A., Soci, C., Abdalla, S., Abellan, X., Balsamo, G., Bechtold, P., Biavati, G., Bidlot, J., Bonavita, M., De Chiara, G., Dahlgren, P., Dee, D., Diamantakis, M., Dragani, R., Flemming, J., Forbes, R., Fuentes, M., Geer, A., Haimberger, L., Healy, S., Hogan, R. J., Hólm, E., Janisková, M., Keeley, S., Laloyaux, P., Lopez, P., Lupu, C., Radnoti, G., de Rosnay, P., Rozum, I., Vamborg, F., Villaume, S., and Thépaut, J.-N.: The ERA5 global reanalysis, Q. J. Roy. Meteor. Soc., 146, 1999–2049, https://doi.org/10.1002/qj.3803, 2020.
Hobbs, L., Cottier, F. R., Last, K. S., and Berge, J.: Pan-Arctic diel vertical migration during the polar night, Mar. Ecol. Prog. Ser., 605, 61–72, https://doi.org/10.3354/meps12753, 2018.
Hobbs, L., Banas, N. S., Cohen, J. H., Cottier, F. R., Berge, J., and Varpe, Ø.: A marine zooplankton community vertically structured by light across diel to interannual timescales, Biol. Lett., 17, 20200810, https://doi.org/10.1098/rsbl.2020.0810, 2021.
Hölemann, J. A., Kirillov, S., Klagge, T., Novikhin, A., Kassens H., and Timokhov, L.: Near-bottom water warming in the Laptev Sea in response to atmospheric and sea-ice conditions in 2007, Polar Res., 30, 6425, https://doi.org/10.3402/polar.v30i0.6425, 2011.
Ito, M., Fukamachi, Y., Ohshima, K. I., and Shirasawa, K.: Observational evidence of supercooling and frazil ice formation throughout the water column in a coastal polynya in the Sea of Okhotsk, Cont. Shelf Res., 196, 104072, https://doi.org/10.1016/j.csr.2020.104072, 2020.
Jakobsson, M., Cherkis, N., Woodward, J., Macnab, R., and Coakley, B.: New grid of Arctic bathymetry aids scientists and mapmakers, EOS, Transactions of the American Geophysical Union, 81, 89–96, https://doi.org/10.1029/00EO00059, 2000.
Johnson, M. A. and Eicken, H.: Estimating Arctic sea-ice freeze-up and break-up from the satellite record: A comparison of different approaches in the Chukchi and Beaufort Seas, Elem. Sci. Anth., 4, 000124, https://doi.org/10.12952/journal.elementa.000124, 2016.
Jönsson, M., Varpe, Ø, Kozłowski, T., Berge, J., and Kröger, R. H. H.: Differences in lens optical plasticity in two gadoid fishes meeting in the Arctic, J. Comp. Physiol. A, 200, 949–957, https://doi.org/10.1007/s00359-014-0941-z, 2014.
Kassens, H.: The Eurasian shelf seas in the Arctic's changing environment: frontal zones and polynya systems in the Laptev Sea, 10th Workshop on Russian-German Cooperation: Laptev Sea System, Kiel, Germany, 17–20 December 2012, Abstracts volume, 5–6, https://oceanrep.geomar.de/id/eprint/20220 (last access: 12 December 2024), 2012.
Kelly, T. B., Davison, P. C., Goericke, R., Landry, M. R., Ohman, M. D., and Stukel, M. R.: The importance of mesozooplankton diel vertical migration for sustaining a mesopelagic food web, Front. Mar. Sci., 6, 508, https://doi.org/10.3389/fmars.2019.00508, 2019.
Khanal, S. and Wang, Z.: Uncertainties in MODIS-Based Cloud Liquid Water Path Retrievals at High Latitudes Due to Mixed-Phase Clouds and Cloud Top Height Inhomogeneity, J. Geophys. Res.-Atmos., 123, 11154–11172, https://doi.org/10.1029/2018JD028558, 2018.
Kirillov, S. A., Dmitrenko, I. A., Hölemann, J. A., Kassens, H., and Bloshkina, E.: The penetrative mixing in the Laptev Sea coastal polynya pycnocline layer, Con. Shelf Res., 63, 34–42, https://doi.org/10.1016/j.csr.2013.04.040, 2013.
Kosobokova, K. N., Hanssen, H., Hirche, H.-J., and Knickmeier, K.: Composition and Distribution of Zooplankton in the Laptev Sea and Adjacent Nansen Basin During Summer, Polar Biol., 19, 63–76, https://doi.org/10.1007/s003000050216, 1998.
Langbehn, T. J. and Varpe, Ø.: Sea-ice loss boosts visual search: Fish foraging and changing pelagic interactions in polar oceans, Glob. Change Biol., 23, 5318–5330, https://doi.org/10.1111/gcb.13797, 2017.
Last, K. S., Hobbs, L., Berge, J., Brierley, A. S., and Cottier, F.: Moonlight drives ocean-scale mass vertical migration of the zooplankton during the Arctic Winter, Curr. Biol., 26, 244–251, https://doi.org/10.1016/j.cub.2015.11.038, 2016.
Leise, T. L., Indic, P., Paul, M. J., and Schwartz, W. J.: Wavelet meets actogram, J. Biol. Rhythms, 28, 62–68, https://doi.org/10.1177/0748730412468693, 2013.
Lemon, D. D., Gower, J. F. R., and Clarke, M. R.: The acoustic water column profiler: a tool for long-term monitoring of zooplankton populations, in: Proceedings of the MTS/IEEE Oceans 2001, An Ocean Odyssey Conference, Proceedings IEEE Cat. No.01CH37295, Vol. 3, 5–8 November 2001, Honolulu, HI, USA, 1904–1909. https://doi.org/10.1109/OCEANS.2001.968137, 2001.
Lemon, D. D., Billenness, D., and Buermans, J.: Comparison of acoustic measurements of zooplankton populations using an Acoustic Water Column Profiler and an ADCP, in: Proceedings of the OCEANS 2008, IEEE Xplore, 15–18 September 2008, Quebec City, QC, Canada, 1–8, https://doi.org/10.1109/OCEANS.2008.5152009, 2008.
Lorke, A., Mcginnis, D. F., Spaak, P., and Wüest, A.: Acoustic observations of zooplankton in lakes using a Doppler current profiler, Freshw. Biol., 49, 1280–1292, https://doi.org/10.1111/j.1365-2427.2004.01267.x, 2004.
Ludvigsen, M., Berge, J., Geoffroy, M., Cohen, J. H., De La Torre, P. R., Nornes, S. M., Singh, H., Sørensen, A. J., Daase, M., and Johnsen, G.: Use of an Autonomous Surface Vehicle reveals small-scale diel vertical migrations of zooplankton and susceptibility to light pollution under low solar irradiance, Sci. Adv, 4, eaap9887, https://doi.org/10.1126/sciadv.aap9887, 2018.
Miller, G. S.: Mysis Vertical Migration in Grand Traverse Bay, Lake Michigan, Observed by an Acoustic Doppler Current Profiler, J. Great Lakes Res., 29, 427–435, https://doi.org/10.1016/S0380-1330(03)70448-1, 2003.
Mol, J., Thomas, H., Myers, P. G., Hu, X., and Mucci, A.: Inorganic carbon fluxes on the Mackenzie Shelf of the Beaufort Sea, Biogeosciences, 15, 1011–1027, https://doi.org/10.5194/bg-15-1011-2018, 2018.
Mulligan, R. P. and Perrie, W.: Circulation and structure of the Mackenzie River plume in the coastal Arctic Ocean, Cont. Shelf Res., 177, 59–68, https://doi.org/10.1016/j.csr.2019.03.006, 2019.
Mulligan, R. P., Perrie, W., and Solomon, S.: Dynamics of the Mackenzie River plume on the inner Beaufort shelf during an open water period in summer, Estuarine, Coast. Shelf Sci., 89, 214–220, https://doi.org/10.1016/j.ecss.2010.06.010, 2010.
Mullison, J.: Backscatter Estimation Using Broadband Acoustic Doppler Current Profilers – Updated, in: Proceedings of the ASCE Hydraulic Measurements & Experimental Methods Conference, Durham, NH, USA, 9–12 July, 1–5, https://www.teledynemarine.com/en-us/support/SiteAssets/RDI/Technical Notes/FSA Documents/FSA031.pdf (last access: 11 December 2024), 2017.
Ochoa, J., Maske, H., Sheinbaum, J., and Candela, J.: Diel and lunar cycles of vertical migration extending to below 1000 m in the ocean and the vertical connectivity of depth-tiered populations, Limnol. Oceanogr.-Meth., 58, 1207–1214, https://doi.org/10.4319/lo.2013.58.4.1207, 2013.
Ofek, E. O.: MATLAB Package for Astronomy and Astrophysics, Astrophysics Source Code Library, bibcode: 2014ascl.soft07005O, https://ui.adsabs.harvard.edu/abs/2014ascl.soft07005O (last access: 11 December 2024), 2014.
Onarheim, I. H., Eldevik, T., Smedsrud, L. H., and Stroeve, J. C.: Seasonal and Regional Manifestation of Arctic Sea Ice Loss, J. Climate, 31, 4917–4932, https://doi.org/10.1175/JCLI-D-17-0427.1, 2018.
Pasternak, A., Drits, A., Arashkevich, E., and Flint, M.: Differential Impact of the Khatanga and Lena (Laptev Sea) Runoff on the Distribution and Grazing of Zooplankton, Front. Mar. Sci., 9, 881383, https://doi.org/10.3389/fmars.2022.881383, 2022.
Perovich, D. K.: The optical properties of sea ice, CRREL Monogr. 96–1, 25 pp., https://apps.dtic.mil/sti/tr/pdf/ADA310586.pdf (last access: 11 December 2024), 1996.
Petrusevich, V., Dmitrenko, I. A., Kirillov, S. A., Rysgaard, S., Falk-Petersen, S., Barber, D. G., Boone, W., and Ehn, J. K.: Wintertime water dynamics and moonlight disruption of the acoustic backscatter diurnal signal in an ice-covered Northeast Greenland fjord, J. Geophys. Res.-Oceans, 121, 4804–4818, https://doi.org/10.1002/2016JC011703, 2016.
Petrusevich, V. Y., Dmitrenko, I. A., Niemi, A., Kirillov, S. A., Kamula, C. M., Kuzyk, Z. Z. A., Barber, D. G., and Ehn, J. K.: Impact of tidal dynamics on diel vertical migration of zooplankton in Hudson Bay, Ocean Sci., 16, 337–353, https://doi.org/10.5194/os-16-337-2020, 2020.
Pivovarov, S. V., Hölemann, J. A., Kassens, H., Antonow, M., and Dmitrenko, I.: Dissolved oxygen, silicon, phosphorous and suspended matter concentrations during the spring breakup of the Lena River, in: Land-Ocean Systems in the Siberian Arctic: Dynamics and History, edited by: Kassens, H., Bauch, H. A., Dmitrenko, I. A., Eicken, H., Hubberten, H.-W., Melles, M., Thiede, J., and Timokhov, L. A., Springer-Verlag, Berlin, Germany, 251–264, https://doi.org/10.1007/978-3-642-60134-7_23, 1999.
Platnick, S., King, M., and Wind, G.: MODIS Atmosphere L2 Cloud Product (06_L2), NASA MODIS Adaptive Processing System, Goddard Space Flight Center, USA [data set], https://doi.org/10.5067/MODIS/MYD06_L2.061, 2017.
Plueddemann, A. J. and Pinkel, R.: Characterization of the patterns of diel migration using a Doppler sonar, Deep-Sea Res. Pt. I, 36, 509–530, https://doi.org/10.1016/0198-0149(89)90003-4, 1989.
Preußer, A., Heinemann, G., Willmes, S., and Paul, S.: Circumpolar polynya regions and ice production in the Arctic: results from MODIS thermal infrared imagery from 2002/2003 to 2014/2015 with a regional focus on the Laptev Sea, The Cryosphere, 10, 3021–3042, https://doi.org/10.5194/tc-10-3021-2016, 2016.
Preußer, A., Ohshima, K. I., Iwamoto, K., Willmes, S., and Heinemann, G.: Retrieval of wintertime sea ice production in Arctic polynyas using thermal infrared and passive microwave remote sensing data, J. Geophys. Res.-Oceans, 124, 5503–5528, https://doi.org/10.1029/2019JC014976, 2019.
Preußer, A., Heinemann, G., Schefczyk, L., and Willmes, S.: A Model-Based Temperature Adjustment Scheme for Wintertime Sea-Ice Production Retrievals from MODIS, Remote Sens., 14, 2036, https://doi.org/10.3390/rs14092036, 2022.
Reiser, F., Willmes, S., and Heinemann, G.: A New Algorithm for Daily sea-ice Lead Identification in the Arctic and Antarctic Winter from Thermal-Infrared Satellite Imagery, Remote Sens., 12, 1957, https://doi.org/10.3390/rs12121957, 2020.
Riggs, G. and Hall, D.: MODIS sea-ice Products User Guide to Collection 6, National Snow and Ice Data Center, University of Colorado: Boulder, CO, USA, https://nsidc.org/sites/default/files/modis-sea-ice-user-guide-c65b15d.pdf (last access: 22 October 2024), 2015.
Rood, S. B., Kaluthota, S., Philipsen, L. J., Rood, N. J., and Zanewich, K. P.: Increasing discharge from the Mackenzie River system to the Arctic Ocean, Hydrol. Process., 31, 150–160, https://doi.org/10.1002/hyp.10986, 2017.
Runge, J. A. and Ingram, R. G.: Under-ice feeding and diel migration by the planktonic copepods Calanus glacialis and Pseudocalanus minutus in relation to the ice algal production cycle in southeastern Hudson Bay, Canada, Mar. Biol., 108, 217–225, https://doi.org/10.1007/BF01344336, 1991.
Sévigny, C., Gratton, Y. and Galbraith, P. S.: Frontal structures associated with coastal upwelling and ice-edge subduction events in southern Beaufort Sea during the Canadian Arctic Shelf Exchange Study, J. Geophys. Res.-Oceans, 120, 2523–2539, https://doi.org/10.1002/2014JC010641, 2015.
Schweiger, A., Lindsay, R., Zhang, J., Steele, M., Stern, H., and Kwok, R.: Uncertainty in modeled Arctic sea ice volume, J. Geophys. Res.-Oceans, 116, C00D06, https://doi.org/10.1029/2011JC007084, 2011.
Sharma, G. D.: Beaufort Sea Shelf, in: The Alaskan Shelf. Hydrographic, Sedimentary, and Geochemical Environment, 418–433, Springer, New York, NY, USA, https://doi.org/10.1007/978-1-4612-6194-0_12, 1979.
Skjoldal, H. R. and Aarflot, J. M.: Abundance and biomass of copepods and cladocerans in Atlantic and Arctic domains of the Barents Sea ecosystem, J. Plankton Res., 45, 870–884, https://doi.org/10.1093/plankt/fbad043, 2023.
Smith, M. and Rigby, B.: Distribution of polynyas in the Canadian Arctic, in: Polynyas in the Canadian Arctic, edited by: Stirling, I. and Cleator, H., 7–28, Canadian Wildlife Service, Occasional Paper No. 45, Ottawa, https://publications.gc.ca/collections/collection_2018/eccc/CW69-1-45-eng.pdf (last access: 22 October 2024), 1981.
Søreide J. E., Dmoch, K., Blachowiak-Samolyk, K., Trudnowska, E., and Daase, M.: Seasonal mesozooplankton patterns and timing of life history events in high-arctic fjord environments, Front. Mar. Sci., 9, 933461, https://doi.org/10.3389/fmars.2022.933461, 2022.
Stanton, T. K., Wiebe, P. H., Chu, D., Benfield, M. C., Scanlon, L., Martin, L., and Eastwood, R. L.: On acoustic estimates of zooplankton biomass, ICES J. Mar. Sci., 51, 505–512, https://doi.org/10.1006/jmsc.1994.1051, 1994.
Stirling, I.: The Biological Importance of Polynyas in the Canadian Arctic, Arctic, 33, 221–381, https://doi.org/10.14430/arctic2563, 1980.
Transdrift: Data from: TRANSDRIFT XIV. OSIS Ocean Science Information System for Expeditions, Numeric Models, Experiments, Leibniz Institute of Marine Sciences at Kiel University, Kiel, Germany, https://portal.geomar.de/metadata/leg/show/324296 (last access: 22 October 2024), 2007.
Transdrift: TRANSDRIFT XII, OSIS Ocean Science Information System for Expeditions, Numeric Models, Experiments, Leibniz Institute of Marine Sciences at Kiel University, Kiel, Germany, https://portal.geomar.de/metadata/cruise/show/317984 (last access: 22 October 2024), 2008.
Tremblay, J. E., Gratton, Y., Fauchot, J., and Price, N. M.: Climatic and oceanic forcing of new, net, and diatom production in the North Water, Deep-Sea Res. Pt. II, 49, 4927–4946, https://doi.org/10.1016/S0967-0645(02)00171-6, 2002.
Varpe, Ø., Daase, M., and Kristiansen, T.: A fish-eye view on the new Arctic lightscape, ICES J. Mar. Sci., 72, 2532– 2538, https://doi.org/10.1093/icesjms/fsv129, 2015.
Vestheim, H., Brucet, S., and Kaartvedt, S.: Vertical distribution, feeding and vulnerability to tactile predation in Metridia longa (Copepoda, Calanoida), Mar. Biol. Res., 9, 949–957, https://doi.org/10.1080/17451000.2013.793806, 2013.
Vestheim, H., Røstad, A., Klevjer, T. A., Solberg, I., and Kaartvedt, S.: Vertical distribution and diel vertical migration of krill beneath snow-covered ice and in ice-free waters, J. Plankton Res., 36, 503–512, https://doi.org/10.1093/plankt/fbt112, 2014.
Wallace, M. I., Cottier, F. R., Berge, J., Tarling, G. A., Griffiths, C., and Brierley, A. S.: Comparison of zooplankton vertical migration in an ice-free and a seasonally ice-covered Arctic fjord: an insight into the influence of sea ice cover on zooplankton behaviour, Limnol. Oceanogr., 55, 831–845, https://doi.org/10.4319/lo.2010.55.2.0831, 2010.
Walsh, J. E., Chapman, W. L., Fetterer, F., and Stewart, S.: Gridded Monthly Sea Ice Extent and Concentration, 1850 Onward, Version 2, National Snow and Ice Data Center, Boulder, CO, USA, [data set], https://doi.org/10.7265/jj4s-tq79, 2019.
Wegner, C., Hölemann, J. A., Dmitrenko, I., Kirillov, S., and Kassens, H.: Seasonal variations in Arctic sediment dynamics – evidence from 1-year records in the Laptev Sea (Siberian Arctic), Global Planet. Change, 48, 126–140, https://doi.org/10.1016/j.gloplacha.2004.12.009, 2005.
Williams, W. and Carmack, E.: Combined effect of wind-forcing and isobath divergence on upwelling at Cape Bathurst, Beaufort Sea, J. Mar. Res., 66, 645–663, https://doi.org/10.1357/002224008787536808, 2008.
Willmes, S. and Heinemann, G.: Pan-Arctic lead detection from MODIS thermal infrared imagery, Ann. Glaciol., 56, 29–37, https://doi.org/10.3189/2015AoG69A615, 2015.
Willmes, S. and Heinemann, G.: Sea-Ice Wintertime Lead Frequencies and Regional Characteristics in the Arctic, 2003–2015, Remote Sens., 8, 4, https://doi.org/10.3390/rs8010004, 2016.
Willmes, S., Krumpen, T., Adams, S., Rabenstein, L., Haas, C., Hoelemann, J., Hendricks, S., and Heinemann, G.: Cross-validation of polynya monitoring methods from multisensory satellite and airborne data: a case study for the Laptev Sea, Can. J. Remote Sens., 36, S196–S210, https://doi.org/10.5589/m10-012, 2010.
Willmes, S., Adams, S., Schröder, D., and Heinemann, G.: Spatio-temporal variability of polynya dynamics and ice production in the Laptev Sea between the winters of 1979/80 and 2007/08, Polar Res., 30, 5971, https://doi.org/10.3402/polar.v30i0.5971, 2011.
Wood, T. M. and Gartner, J. W.: Use of acoustic backscatter and vertical velocity to estimate concentration and dynamics of suspended solids in Upper Klamath Lake, south-central Oregon: Implications for Aphanizomenon flos-aquae, USGS Scientific Investigations Report 5203, 1–20, https://doi.org/10.3133/sir20105203, 2010.
Yang, D., Kane, D. L., Hinzman, L., Zhang, X., Zhang, T., and Ye, H.: Siberian Lena River hydrologic regime and recent change, J. Geophys. Res.-Atmos., 107, 4694, https://doi.org/10.1029/2002JD002542, 2002.
Yang, D., Shi, X., and Marsh, P.: Variability and extreme of Mackenzie River daily discharge during 1973–2011, Quatern. Int., 380–381, 159–168, https://doi.org/10.1016/j.quaint.2014.09.023, 2015.
Zhang, J. L. and Rothrock, D. A.: Modeling global sea ice with a thickness and enthalpy distribution model in generalized curvilinear coordinates, Mon. Weather Rev., 131, 845–861, https://doi.org/10.1175/1520-0493(2003)131<0845:MGSIWA>2.0.CO;2, 2003.