the Creative Commons Attribution 4.0 License.
the Creative Commons Attribution 4.0 License.
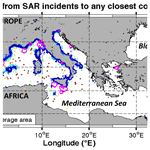
Coastal high-frequency radars in the Mediterranean – Part 2: Applications in support of science priorities and societal needs
Eva Aguiar
Michele Bendoni
Maristella Berta
Carlo Brandini
Alejandro Cáceres-Euse
Fulvio Capodici
Vanessa Cardin
Daniela Cianelli
Giuseppe Ciraolo
Lorenzo Corgnati
Vlado Dadić
Bartolomeo Doronzo
Aldo Drago
Dylan Dumas
Pierpaolo Falco
Maria Fattorini
Maria J. Fernandes
Adam Gauci
Roberto Gómez
Annalisa Griffa
Charles-Antoine Guérin
Ismael Hernández-Carrasco
Jaime Hernández-Lasheras
Matjaž Ličer
Pablo Lorente
Marcello G. Magaldi
Carlo Mantovani
Hrvoje Mihanović
Anne Molcard
Baptiste Mourre
Adèle Révelard
Catalina Reyes-Suárez
Simona Saviano
Roberta Sciascia
Stefano Taddei
Joaquín Tintoré
Yaron Toledo
Marco Uttieri
Ivica Vilibić
Enrico Zambianchi
Alejandro Orfila
The Mediterranean Sea is a prominent climate-change hot spot, with many socioeconomically vital coastal areas being the most vulnerable targets for maritime safety, diverse met-ocean hazards and marine pollution. Providing an unprecedented spatial and temporal resolution at wide coastal areas, high-frequency radars (HFRs) have been steadily gaining recognition as an effective land-based remote sensing technology for continuous monitoring of the surface circulation, increasingly waves and occasionally winds. HFR measurements have boosted the thorough scientific knowledge of coastal processes, also fostering a broad range of applications, which has promoted their integration in coastal ocean observing systems worldwide, with more than half of the European sites located in the Mediterranean coastal areas. In this work, we present a review of existing HFR data multidisciplinary science-based applications in the Mediterranean Sea, primarily focused on meeting end-user and science-driven requirements, addressing regional challenges in three main topics: (i) maritime safety, (ii) extreme hazards and (iii) environmental transport process. Additionally, the HFR observing and monitoring regional capabilities in the Mediterranean coastal areas required to underpin the underlying science and the further development of applications are also analyzed. The outcome of this assessment has allowed us to provide a set of recommendations for future improvement prospects to maximize the contribution to extending science-based HFR products into societally relevant downstream services to support blue growth in the Mediterranean coastal areas, helping to meet the UN's Decade of Ocean Science for Sustainable Development and the EU's Green Deal goals.
- Article
(12757 KB) - Full-text XML
- Companion paper
-
Supplement
(204 KB) - BibTeX
- EndNote
The coastal and ocean economy has been since ancient times and is now, more than ever, the backbone of the Mediterranean countries' blue economy. In 2017, the Mediterranean was the third largest sea basin in terms of gross value added (GVA) and the first in terms of employment (European Commission, 2020). The key sector is clearly coastal tourism, from which the Mediterranean is the world's leading destination, followed by maritime transport, living resources and port activities. Furthermore, coastal tourism and fisheries benefit from the location of the marine protected areas (MPAs), which currently cover 7 % of the northern Mediterranean Sea surface (Meola et al., 2019) and are expected to increase as one of the goals of the United Nations (UN) Decade of Ocean Science for Sustainable Development.
Mediterranean coastal areas and communities are, however, negatively impacted by all human activities related to these traditional sectors. Regarding the sector of maritime transport, it is worth highlighting that the Mediterranean Sea is one of the world's busiest shipping lanes of oil and gas tankers, container vessels, and ships, involving a higher risk of marine oil and marine litter (ML) pollution. Although the extent of the latter is not fully understood yet, first estimations provided from Cózar et al. (2015) identify the Mediterranean Sea as a great accumulation zone of plastic debris comparable to the accumulation zones described for the five subtropical ocean gyres. Additionally, Soto-Navarro et al. (2021) have recently found that the hot spots for the ML risk concentrate in the coastal regions, highly impacting Mediterranean biodiversity, especially in the MPAs and particularly in those near ML sources.
Given the strategic role of ports in the globalized trading system, it is important to underline that four ports from the Mediterranean (i.e., Algeciras, Valencia, Piraeus and Marseille) are included in the top-five European ports when looking at different categories according to the Eurostat statistics from 2020. Moreover, the Mediterranean Sea hosts the three main migratory routes to Europe, representing a huge humanitarian, political and security challenge for the bordering countries. In this context, we cannot ignore the more than 99 400 migrants who arrived in Europe in 2020, mainly by sea and particularly to Spain, Greece and Italy by crossing the Mediterranean Sea according to the data from the International Organization for Migration (IOM). This complex migratory hub contributes to the increased risk to life and maritime safety in the Mediterranean.
Last but not least, as recently reviewed by Tintoré et al. (2019), the Mediterranean is one of the most vulnerable regions in the world due to the impact of climate change. As a result of large-scale warming, among many other impacts reported by the authors, an increase in frequency and/or intensity of extreme events is expected (Mitchell et al., 2006). In this context, De Alfonso et al. (2021) points out an average of eight storms per year registered for the Spanish Mediterranean coast with particular severe events registered in November 2001 (Gómez et al., 2002), October 2007 (Cohuet et al., 2011), December 2008 (Sánchez-Arcilla et al., 2014), January 2017 and January 2020 (Amores et al., 2020; de Alfonso et al., 2021, Lorente et al., 2021; Sotillo et al., 2021). Aiming to monitor and understand this regional and sub-regional ocean state and variability from daily to interannual scales, a set of indicators for the Mediterranean Sea and the Balearic Islands (Juza and Tintoré, 2021) are made available through a user-friendly visualization tool by SOCIB (Tintoré et al., 2013, 2019).
The increased capability to address the abovementioned regional challenges at the required spatiotemporal scales has directly benefited, inter alia, from the key features of high-frequency radar (HFR hereinafter) technology, i.e., unprecedentedly high spatiotemporal resolution (i.e., 0.2–6 km and 15–60 min) over wide coastal areas (up to 200 km offshore, depending on the operational frequency). HFRs provide continuous monitoring of the surface circulation (Lipa, Barrick and Maresca, 1981; Paduan and Graber, 1997; Headrick and Thomason, 1998; Molcard et al., 2009; Paduan and Washburn, 2013; Wyatt, 2014; Roarty et al., 2019; Dumas and Guérin, 2020), increasingly, wave parameters (Lipa et al., 1990, 2005, 2006; Gurgel et al., 2006; Wyatt et al., 2006; Orasi et al., 2018; Wyatt and Green, 2009; Long et al., 2011; Wyatt, 2011; Falco et al., 2016; Saviano et al., 2019, 2020, 2022; Basáñez et al., 2020; Bué et al., 2020) and, occasionally, wind field (Long and Trizna, 1972; Heron, 2002; Huang et al., 2004; Shen et al., 2012; Kirincich et al., 2016a; Zeng et al., 2016, 2018; Shen and Gurgel, 2018; Saviano et al., 2021). This land-based remote sensing technology gives us a unique insight to coastal ocean state and variability with relative ease in terms of technical effort, manpower and costs (i.e., for the same amount of information and compared to other conventional observing platforms), allowing us to improve our understanding of sub-mesoscale and mesoscale coastal processes.
Moreover, coastal ocean surface current and wave real-time information, which represents the primary and secondary basic products of HFRs, respectively, is being used extensively by search and rescue (Ullman et al., 2006; Ličer et al., 2020; Révelard et al., 2021), environmental agencies for pollutant monitoring of oil spills (Abascal et al., 2009), marine litter tracking (Declerck et al., 2019), recreational activities, navigational safety, ports and shipping, ship detection and tracking (Ponsford et al., 2001; Dzvonkovskaya et al., 2007; Maresca et al., 2013; Laws et al., 2016), coastal and offshore engineering applications, aquaculture, marine renewables (Wyatt, 2012; Basáñez and Pérez-Muñunzuri, 2021; Mundaca-Moraga et al., 2021), and early warning detection systems for natural hazards (Lipa et al., 2006; Gurgel et al., 2011; Grilli et al., 2015; Guérin et al., 2018), among others. Furthermore, the mapping of surface currents at high spatiotemporal resolution provided by the HFRs in the coastal strip allow us to use them as a ground truth for coastal model real-time assessment (Wilkin and Hunter, 2013; Lorente et al., 2016, 2019b; Mourre et al., 2018; Aguiar et al., 2020) and improvement through HFR data assimilation (Breivik and Saetra, 2001; Paduan and Shulman, 2004; Barth et al., 2008; Iermano et al., 2016; Hernández-Lasheras et al., 2021), as well as for the evaluation of coastal remote sensing products (Manso-Narvarte et al., 2018; Caballero et al., 2020; Gommenginger et al., 2021). The development of advanced HFR data products such as gap-filled nowcasts and Lagrangian trajectories allows us to satisfactorily estimate transport, making HFR data a key asset in the assessment and protection of the coastal marine environment, including dispersal and retention of particles (Cianelli et al., 2017; Hernández-Carrasco et al., 2018a; Davila et al., 2021), cross-shelf exchanges and transport (Sciascia et al., 2018), eddy tracking (Nencioli et al., 2010; Bagaglini et al., 2020), and 3D eddy characterization (Manso-Narvarte et al., 2021).
In addition to this, many strong coordinated efforts to significantly increase the prompt distribution, availability, easy access and accuracy of HFR data have been made in recent years at the global (Roarty et al., 2019), European (Rubio et al., 2017) and regional levels (Lorente et al., 2022), also leveraged by national initiatives and specific projects. These joint efforts have enhanced the creation of a community at the HFR operator level, therefore accelerating the speed of the take-up of data, also underpinning the growth of HFR multidisciplinary applications worldwide (Fujii et al., 2013; Paduan and Washburn, 2013; Wyatt, 2014; Rubio et al., 2017 and Roarty et al., 2019).
This broad range of applications has also boosted the positive trend in HFR installation all around the world. Consistently, HFRs are also currently playing a crucial role as one of the backbones of the coastal ocean observing systems – COOSs – of the Mediterranean Sea, which currently encompass more than half of the existing HFR systems installed in Europe (Lorente et al., 2022), therefore constituting an important focus of HFR activity.
Demonstrating the potential of the HFR observing and monitoring regional capabilities, this work reviews the existing mature and emerging scientific and societal applications using HFR data, developed to address the major challenges identified in the Mediterranean coastal waters, organized around three main topics: (i) maritime safety, (ii) extreme hazards and (iii) environmental transport processes. Also recognizing the added value of networking, it is worth highlighting that this review encompasses the main outcomes of multidisciplinary, international and intersectoral regional coordinated efforts in the framework of the Mediterranean Operational Network for the Global Ocean Observing System (MONGOOS) HFR Task Team. These endeavors are primarily focused on meeting end-user and science-driven requirements, aiming to unlock HFR data potential and deliver greater uptake, use and value from the data for the benefit of ecosystems, services and human activities of the coastal areas of the Mediterranean Sea.
This paper constitutes the second part of two complementary contributions, the first one providing a detailed overview of the main achievements, ongoing activities, future challenges and the roadmap towards an integrated, mature HFR network in the Mediterranean Sea (Lorente et al., 2022). The sections of this paper are as follows: Sect. 2 presents several HFR applications addressing science priorities and societal needs, classified in the abovementioned three topics. Section 3 includes the discussion and a preliminary assessment of the capabilities of the existing HFR applications. Based on this assessment, Sect. 4 outlines the future prospects for HFR applications along with a set of key recommendations aiming to leverage the HFR data to their fullest extent, thus helping to harness HFR potential in the further development of operational monitoring systems at the regional level.
This contribution will help to achieve the goals of the United Nations (UN) Decade of Ocean Science for Sustainable Development (Ryabinin et al., 2019) and to address the transitional changes required towards the European Green Deal (Sikora, 2021). Finally, a summary and the main conclusions are provided in Sect. 5.
This section presents the existing advanced and emerging scientific and societal applications using HFR data, aiming to address science priorities and societal needs identified in the Mediterranean coastal waters (Lorente et al., 2022), organized around three main topics: (i) maritime safety, (ii) extreme hazards and (iii) environmental transport processes.
2.1 Maritime safety
Around 200 000 large vessels operate annually in the Mediterranean Sea, including ferries as well as cargo and commercial vessels, among which around 300 tankers transport oil-based products every day, accounting for more than 350 million tons per year (more than 25 % of the world's oil tonnage) as highlighted by Di Muccio et al. (2020). This intense maritime traffic makes the basin a susceptible area in terms of oil spills, search and rescue (SAR) operations, and other maritime emergencies. Over the past half-century spills over the sea from tankers have shown a downward trend, reaching the lowest number in early 2020 due to the global health and economic crisis triggered by the COVID-19 pandemic (March et al., 2021). However, oil spills as well as chemical spills and other hazardous substance releases are still present, putting marine health at risk. For instance, in the second half of February 2021, around 170 km of coastline from Israel to southern Lebanon suffered from a large oil spill (García-Sánchez et al., 2022), one of the worst ecological disasters in decades. In this context, accurate forecasting of oil spill modeling (for this particular event, the model MEDSLIK-II was used, as described in De Dominicis et al., 2013a, b) and Lagrangian trajectory analysis of floating objects (Sayol et al., 2014; Ličer et al., 2020) have been demonstrated to successfully help marine SAR operations and oil spill containment. These forecasts depend strongly on the accuracy of the forcing data (i.e., wind, waves and currents, as stated in Sect. 2.1.2) ingested in atmospheric and oceanographic models, for which ocean surface current maps from HFRs in particular can greatly improve short-term model outputs due their high resolution and their near-real-time nature (Abascal et al., 2009, 2012; Breivik et al., 2013), as described in Sect. 2.1.3. In this context real-time HFR data were accepted as a reliable operational tool for SAR, oil spill and other operational protocols in coastal waters (Roarty et al., 2019). Concerning the last two sections, it is important to mention the wider implementation of ocean models and short-term predictions in other crosscutting areas from the three addressed main topics. Nevertheless, we have included them in this section to highlight the HFR strengths for SAR applications through model assessment and improvement, backtracking, and short-term forecasting.
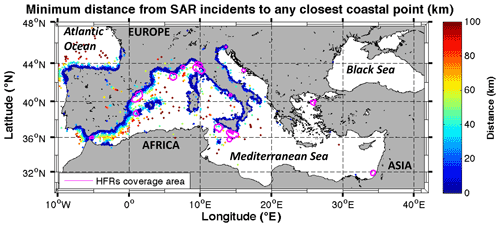
Figure 1Map of the Mediterranean showing the HFR mean spatial coverage (pink contours) and the location of SAR incidents of France, Italy, Slovenia and Spain from 2019 colored based on their distance to the closest coastal point. For further details about the operational status and the names of the HFR systems the reader is referred to Lorente et al. (2022). In order to indicate the detail of the coverage of the HFRs and their proximity to the incident locations in particular areas, Figs. S1, S2 and S3, available in the Supplement, show zoomed-in views of Italy, the northwestern Mediterranean sub-basin and the Strait of Gibraltar, respectively.
2.1.1 Search and rescue
Agencies in charge of SAR operations, marine pollution response and maritime traffic control are among the most significantly targeted users of reliable met-ocean information. Access to multi-platform quality-controlled near-real-time met-ocean observations and high-resolution forecasts available for their specific areas of responsibility for marine SAR, assigned by the IMO (International Maritime Organization), is essential for them to support emergency response missions. Wind, wave and surface current observations and forecasts are needed to be seamlessly integrated into their SAR emergency tools in order to predict the trajectory of a drifting target for determining the optimal search region. In the sphere of maritime safety, HFRs have the great advantage of providing high-spatiotemporal-resolution surface currents in wide coastal areas very close to the coastline when HFR gap-filling methods are applied (listed in Sect. 2.1.3) and where most of the SAR incidents occur (as shown by Fig. 1), as the review of the location of the SAR incidents from five countries (i.e., Croatia, France, Italy, Slovenia and Spain) along 2019 and from Malta along 2020 clearly shows.
- i.
Croatia. A total of 612 SAR interventions were registered in 2019, 389 of which are SAR interventions and 223 are MEDEVAC ones (i.e., actions related to transportation of injured or sick persons). Most of these incidents occur during summer from June to September and over 98 % in inner and territorial waters. Coastal waters from Croatia are operationally monitored by the HFR-SPLIT WERA Radar System, consisting of two WERA HFR sites (Ražanj and Stončica) in the eastern part of the eastern mid-Adriatic basin. The HFR-NASCUM system is a historical network located in the eastern part of the Gulf of Venice. All four sites were used to build NEURAL project short-term predictions (described in Sect. 2.1.3).
- ii.
France. France has five SAR responsibility areas in the continental littoral coordinated by 340 SAR operators from the five maritime rescue coordination centers, including one for the French Mediterranean responsibility area. In 2019, a total of 13 507 SAR incidents occurred (with 22 313 people assisted), 51 % of which were from June to September, as indicated on the website of the French Ministry of the Sea. In particular, the number of SAR incidents in the French Mediterranean responsibility area accounts for 23 % (3110) of the total number of cases and 32 % (7293) of the people assisted, as included in the 2019 activity report of the French Mediterranean Coordination Center. A total of 94 % of SAR incidents occur in coastal areas in the first 12 nm (22.2 km) and mostly during summer season (from June to September), with more of the 89 % related to recreational boating and sailing. Currently, two HFR networks are operating in the French Mediterranean coastal waters of Nice and Toulon, named HFR-MedTln and HFR-MedNce.
- iii.
Italy. SAR operations are under the responsibility of the Italian coast guard covering 500 000 km2 of sea and 8000 km of coast. In 2019 the Italian coast guard responded to 1875 SAR missions, 226 of them related to human migration. SkyTruth, a non-governmental agency, reported one spill 60 km south of Genoa in the Ligurian Sea. Six HFR networks are currently monitoring the coastal areas of the Tyrrhenian and Ligurian Sea (HFR-TirLig) as well as the Tuscan Archipelago (HFR-LaMMA), Gulf of Naples (HFR-GoN), the Malta–Sicily Channel (HFR-CALYPSO), and the northern Adriatic Sea and the Gulf of Trieste (HFR-NAdr), with one recently deployed in the southwest of Sicily island (HFR-SIC). Two additional HFR networks in the Gulf of Manfredonia (HFR-GoM) and the Gulf of Venice (HFR-NASCUM) are historical deployments.
- iv.
Malta. There is one maritime rescue coordination center with around 50 SAR operators, covering one search and rescue region (SRR) of 267 874 km2 with 196.8 km of coastline (including Comino and Gozo). During 2020, 429 missions were coordinated by the MRCC (Maritime Rescue Coordination Centre) in Malta, 26 % of which were reported as SAR cases occurring within Maltese territorial seas. The HFR-CALYPSO monitors the Malta–Sicily Channel, accounting for seven HFR sites and the HFR-CALYPSO-SOUTH, and is composed of two HFR sites located in the south of Malta. HFR data are combined with forecast model outputs to get the best representation of the sea state during SAR operations.
- v.
Slovenia. Slovenia has 42 km of coastline and a semi-enclosed coastal area. During 2019, the SAR agency responded to nine SAR missions (seven times the rescue boat went out to sea, while two rescues were of injured people on a moored boat in port). All cases occurred within 3 nm from the coast (i.e., three within 200 m, three around 1 nm and one at 3 nm from the coast). The HFR-NAdr, in the northern Adriatic Sea and the Gulf of Trieste, is jointly operated through transnational collaboration with Italy.
- vi.
Spain. The four SAR responsibility areas cover 1 500 000 km2 of marine surface (3 times the size of the Spanish national territory) and 8000 km of coastline. The Spanish Maritime Safety and Rescue Agency (SASEMAR hereinafter) is divided into 19 MRCCs plus one national center, with more than 370 SAR operators. SASEMAR responded to 5891 missions in 2019, almost 88 % of which were SAR operations. A total of 50 % of the total SAR incidents occurred within 3 km of the Spanish coastlines. Of the seven HFR networks operating inside their four responsibility areas, three of them are located in the western Mediterranean, monitoring the Strait of Gibraltar (HFR-Gibraltar), the Ebro Delta (HFR-Ebro) and the Ibiza Channel (HFR-Ibiza), and all of them are integrated in the SASEMAR Environmental Data Server.
As previously mentioned, maritime SAR operations most often depend on leveraging Lagrangian tracking tools using timely and reliable knowledge of surface circulation, near-surface winds and, if applicable, surface gravity waves. Surface circulation is generally provided by numerical circulation models, but HFR observations can offer valuable insight into marine conditions over the region of the accident and can – especially when coupled to short-term prediction models (see Sect. 2.1.3) – act as a complementary input for Lagrangian predictions, hindcasts or backtracking simulations. Révelard et al. (2021) evaluated the use of HFR-derived trajectories to complement drifter observations for assessing the performance of different models (i.e., GLO-MFC, IBI-MFC and MED-MFC, provided by the Copernicus Marine Service and WMOP) in predicting Lagrangian trajectories. They used the skill score (SS) metric based on the normalized cumulative Lagrangian separation distance (Liu and Weisberg, 2011), which is a commonly used metric for assessing Lagrangian performance. They have concluded that, whereas drifters only provide assessment along their drifting paths, HFR allows for a large number of trajectories, improving not only the robustness of the skill score statistics but also the spatial and temporal assessment of the model performance (Fig. 2). Since HFR data are quasi-continuous in time, this method can be applied in near-real time, which is a strong advantage for evaluating extremely scenario-dependent models. Indeed, the quality of any numerical model performance varies with time and can have substantial fluctuations on short temporal and spatial scales even if the model otherwise exhibits good overall forecasting skills. In cases like these, quality-controlled HFR observations represent particularly valuable short-term inputs for Lagrangian products assisting SAR efforts.
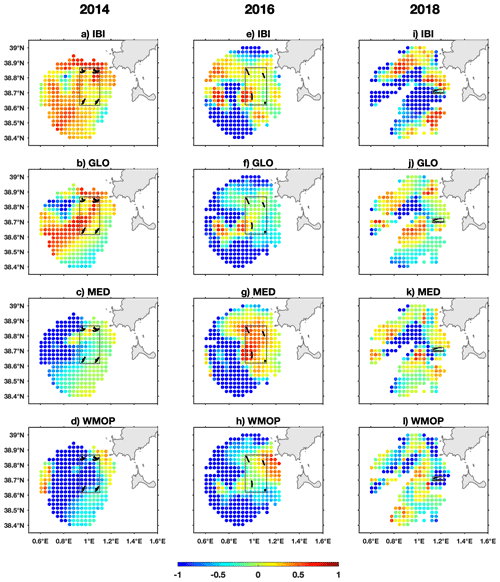
Figure 2Temporally averaged skill score SS∗ obtained for four models as indicated in the title of each panel by comparing against the HFR-derived trajectories of the Ibiza Channel during a forecast time of 6 h. Simulated trajectories are initialized hourly at each grid point on 30 September 2014 from 13:00 to 16:00 (a–d), on 28 July 2016 from 16:00 to 22:00 (e–h), and on 15 November 2018 from 13:00 to 16:00 (i–l). SS∗ values, with red representing the higher average model performance, are only obtained in those grid points with data temporal availability equal to or higher than 80 %. Black lines show the drifter paths available during the same periods, and the boxes indicate the regions where the averages are applied for comparison with the results obtained with drifter observations. Original source: from Révelard et al. (2021).
Aiming to improve the applicability of this model assessment methodology for SAR operations in coastal areas, Révelard et al. (2021) also analyzed the SS sensitivity to different forecast horizons and showed that in coastal regions (i) the SS is sensitive to the forecast time, i.e., the longer the forecast (i.e., 72 h), the higher the SS value, due to the high variability of the surface currents; and (ii) a shorter forecast time (e.g., 6 h), consistent with the duration of the search that maximizes survivors in SAR missions, is therefore more appropriate. In addition, they have shown that, whereas the original definition of the skill score from Liu and Weisberg (2011) is correct for analyzing its spatiotemporal distribution, the use of a novel skill score (SS∗) is recommended to assess the average model performance over an area of interest and along a specified period to avoid biased conclusions. The advantage of the SS∗ is that, despite its similar formulation to the SS defined by Liu and Weisberg (2011), SS∗ does not impose negative values to zero, allowing us to obtain a correct average, as in Fig. 2. However, they clarified that only SS∗ values >0.5 should be interpreted as good agreement between HFR surface current observations and model outputs.
A further academic study of the value of quality-controlled HFR observations in SAR operations was the recent case of a person lost at sea in the northern Adriatic during a Sirocco storm on 29 October 2018. In this case, HFR-NAdr observations were employed for hindcasting and survivor's drift trajectory verification (Ličer et al., 2020). Figure 3 depicts Lagrangian drifter dispersal computed from modeled surface winds (the dominant contribution to the drift in this case) and HFR surface currents from the HFR-NAdr network in the Gulf of Trieste after this accident.
Even though in this case part of the survivor's trajectory outside the HFR-NAdr domain had to be inferred from extrapolated currents, such HFR-based nowcasting products would have been valuable during this and similar rescue attempts. However, since HFR data arrive in near-real time, some sort of model-based extension of their prediction horizon is necessary before they can be used for operational nowcasting. One possible solution is data assimilation of HFR data into a numerical model (see Sect. 2.1.2.), followed by a forecasting time window. An alternative and numerically less demanding option that has gained ground in recent years is the machine-learning approach whereby a neural network model is trained on past data and then used to create short-term predictions of surface currents, as addressed in Sect. 2.1.3.
2.1.2 Model assessment and improvement
This section addresses one of the main interests and needs of end users of operational oceanography information: users want to be able to have confidence in modeled data and they need to know how good they are. Addressing end-user overarching concerns, model assessment, essentially built upon comparison to observations, is crucial to evaluate the quality of the diversity of modeling products available in a systematic and long-term routine manner and to inform users about their usefulness for a given application.
For this reason, also seeking to strengthen end-user loyalty, the validation of operational ocean forecasting systems against independent measurements constitutes a core activity in operational oceanography (Hernández et al., 2015) since it aids in (i) inferring the relative strengths and weaknesses in the modeling of several key physical processes, (ii) comparing different versions of the same operational ocean forecasting system and evaluating potential improvements and degradations before a new version is transitioned into operational status, (iii) comparing coarse-resolution “parent” and nested high-resolution “child” systems to quantify the added value of downscaling, and (iv) informing end users about the consistency and skill of the modeling products disseminated.
Developments in ocean modeling have clearly advanced to address the challenges associated with the increased resolution and its application to coastal areas, also responding to the high demand for providing 4D estimates of multiple oceanic variables at fine scales (Mourre et al., 2018; Fox-Kemper et al., 2019). Coastal modeling faces numerous challenges and issues such as downscaling and representation of open boundary conditions or land–sea and air–sea interactions (Kourafalou et al.et al., 2015a). Synergies between models and ocean observations are needed to face these challenges and improve representation of ocean processes (Kourafalou et al., 2015b; De Mey-Frémaux et al., 2019; Davidson et al., 2019). Additionally, it is worth mentioning the current lack of real-time and historical availability of observations in coastal areas, which limits the operational capability and reduces the potential of skill assessment operational services aiming to provide synthetic metrics addressing specific user needs (Révelard et al., 2021).
Within this context, HFR systems play a first-order role thanks to their unique ability to provide fine-resolution maps of the surface currents over broad coastal areas. This ability of HFR systems makes them particularly appropriate for the validation of numerical models in coastal areas, where other observations are scarce and/or their resolutions (i.e., in space or in time) are not high enough to capture the fine scale. Many HFR systems have therefore been used for this purpose in several regions of the Mediterranean Sea including the Northern Current area off Toulon (Berta et al., 2014a), the Ebro Delta area (Lorente et al., 2016, 2021; Ruiz et al., 2020; Aguiar et al., 2020; Sotillo et al., 2021), the northern Adriatic (Vilibić et al., 2016), the Gulf of Naples (Uttieri et al., 2011), the Ibiza Channel (Mourre et al., 2018; Aguiar et al., 2020; Révelard et al., 2021; Sotillo et al., 2021) and the Strait of Gibraltar (Lorente et al., 2019a; Aguiar et al., 2020).
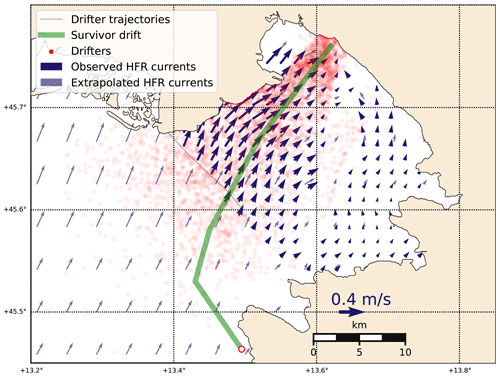
Figure 3Using HFR currents for Lagrangian hindcasting of an accident on 29 October 2018 in the Gulf of Trieste; details in Ličer et al. (2020). Blue arrows denote the HFR surface current field on 29 October 2018 at 22:00 UTC. Thinner (but scaled to length) light blue arrows depict nearest-neighbor extrapolated currents outside the HFR domain (every third point is plotted for clarity). Red dots denote modeled virtual drifter locations after 24 h of the drift, starting from the accident location (white circle at 13.495∘ E, 45.4635∘ N). The green line indicates the survivor's estimate of his drift trajectory. Adapted from Ličer et al. (2020).
An example of this added value of HFR data was recently shown in the multi-model comparison exercise performed in the Strait of Gibraltar in 2017 (Lorente et al., 2019a). In that case, the IBI-MFC model (Sotillo et al., 2015) was compared against their partially nested SAMPA (Sánchez-Garrido et al., 2013) high-resolution coastal forecast system to elucidate the accuracy of each system characterizing the Atlantic Jet (AJ) inflow dynamics. To this aim, HFR-derived hourly currents at the midpoint of the selected transect (square in Fig. 4a) were used as a benchmark. The scatter plot of HFR-derived hourly current speed versus direction (taking as a reference the north and positive angles clockwise) revealed interesting details (Fig. 4b): (i) the AJ flowed predominantly eastwards, forming an angle of 78∘ with respect to the north; (ii) the current velocity, on average, was 1 ms−1 and reached peaks of 2.5 ms−1. Speeds below 0.5 ms−1 were registered along the entire range of directions; (iii) westward currents, albeit in the minority, were also observed and tended to predominantly form an angle of 270∘ (i.e., towards the Atlantic), mostly related to intense easterly winds episodes (Garret, 1983; García-Lafuente et al., 2002; Menemenlis et al., 2007; Péliz et al., 2009; Reyes et al., 2015; Lorente et al., 2019a, b; Bolado-Penagos et al., 2021), as further detailed in Sect. 2.2.1. The scatter plot of SAMPA estimations presented a significant resemblance in terms of prevailing current velocity and direction (Fig. 4c). Although the time-averaged speed and angle were slightly smaller (0.9 ms−1) and greater (88∘), respectively, the main features of the AJ were qualitatively reproduced: maximum velocities (up to 2.5 ms−1) were associated with an eastward flow and an AJ orientation in the range of 50–80∘. Surface flow reversals to the west were also properly captured. By contrast, noticeable differences emerged in the scatter plot of regional IBI-MFC estimations (Fig. 4d): surface current velocities below 0.3 ms−1 were barely replicated, and the AJ inversion was only observed very occasionally. Despite the fact that IBI-MFC appeared to properly portray the mean characteristics of the eastward flow, the model tended to favor flow directions between 60 and 180∘ and to overestimate the current velocity, with averaged and maximum speeds around 1.17 and 2.80 ms−1, respectively.
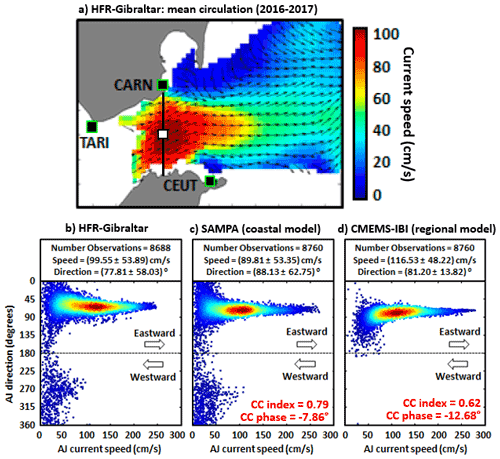
Figure 4(a) HFR-derived mean surface circulation pattern in the Strait of Gibraltar for 2016–2017: classical Atlantic Jet inflow into the Mediterranean, with strong surface currents flowing to the NE. Solid black squares represent radar sites. The black line and the related white square indicate the selected transect and its midpoint, respectively. (b–d) Quantitative validation at the selected grid point (5.43∘ W, 35.99∘ N) within the Strait of Gibraltar: annual (2017) scatter plot of hourly current speed versus direction (angle measured clockwise from the north); estimations provided by HFR-Gibraltar (b), SAMPA high-resolution coastal model (c) and IBI-MFC (d), a regional model into which SAMPA is nested. Mean and standard deviation values of both AJ speed and direction are gathered in black boxes. Magnitudes of the complex correlation (i.e., CC index) and phase between HFR and model-predicted currents are provided in red font. Adapted from Lorente et al. (2019a).
In summary, HFR measurements are able to precisely assess the added value of the downscaling performed through the SAMPA coastal system with respect to the IBI-MFC regional solution in which SAMPA is nested. Overall, a steady improvement in the Atlantic Jet characterization is evidenced in model performance when zooming from regional to coastal configurations, highlighting the benefits of the downscaling approach adopted and also the potential relevance of a variety of factors at local scale, among others: a more refined horizontal resolution, a tailored bathymetry or the higher spatiotemporal resolution of the atmospheric forcing. Furthermore, SAMPA appeared to better reproduce the reversal events detected with HFR estimations, demonstrating the added value of imposing accurate meteorologically driven barotropic velocities in the open boundaries, imported from the NIVMAR storm surge model (Álvarez-Fanjul et al., 2001), in order to consider the remote effect of the atmospheric forcing over the entire Mediterranean basin, which was only partially included in IBI-MFC.
During the next phase of the Copernicus Marine Service, more focus will be on coastal downstream applications (e.g., very high-resolution ocean models integrated with coastal observatories) for a wide range of coastal stakeholders including ports and environmental agencies. Despite the significant progress in the field of coastal modeling, some storm-induced hazards are still not properly resolved (or even misrepresented) by ocean models due to a variety of factors (e.g., horizontal resolution that is too coarse, inadequate meteorological forcing, poor representation of land–sea interactions and the related river freshwater outflows, among others) as described by Sotillo et al. (2021). Within this framework, HFR might act as a monitoring cornerstone to calibrate and validate successive, upgraded versions of operational ocean forecasting models with the aim of better capturing extreme events in terms of strength, extension and timing (Lorente et al., 2021).
Aguiar et al. (2020) used the three HFR systems available in the western Mediterranean Sea (Strait of Gibraltar, Ibiza Channel – described in Tintoré et al., 2013, and Lana et al., 2016 – and Ebro Delta) to evaluate the impact of downscaling on the surface coastal circulation in the case of the Western Mediterranean OPerational forecasting system (WMOP) (Juza et al., 2016; Mourre et al., 2018). The authors showed that the time-average circulation in the coastal areas of the Ebro Delta and Ibiza Channel were improved through downscaling. In particular, the nested model showed a better representation of the small-scale coastal flow intensification at the mouth of the Ebro River and a refinement in the characterization of the circulation in the Ibiza Channel. Notice that HFR-Gibraltar, HFR-Ebro and HFR-Ibiza versus model comparisons are updated daily on the SOCIB WMOP web page https://socib.es/?seccion=modelling&facility=wmedvalidation (last access: 9 May 2022). Those HFR systems, among others, are also integrated in the IBISAR science-based data downstream service (Reyes et al., 2020a) – freely available under registration at https://www.ibisar.es/ (last access: 9 May 2022) – for visualizing, comparing and evaluating the performance of ocean current predictions in the Iberian–Biscay–Irish regional seas. IBISAR allows the identification of the most accurate ocean current dataset in a specific area and period of interest, thus facilitating decision-making for SAR operators and emergency responders. Lorente et al. (2022) consider the IBISAR service to be a successful example of the long-lasting engagement built in collaboration between HFR operators and end users (i.e., the Spanish Maritime Safety and Rescue Agency). Additionally, those HFR systems are also being used for IBI-MFC model assessment purposes by means of the NARVAL multiparameter and multi-platform validation tool (Lorente et al., 2019c) for IBI-MFC model validation.
Another added value of HFR systems is their use to improve model forecast through data assimilation (DA). DA aims at optimally combining observations and models to provide a better representation of the ocean dynamics. In this sense, HFR provides very valuable high-resolution observations in areas where satellite observations tend to suffer from limitations due to the vicinity of the coast (Vignudelli et al., 2019). While the assimilation of HFR measurements has been applied in many regions of the world since the first studies from Breivik (2001) and Oke et al. (2002), only a limited number of studies have been performed in the Mediterranean Sea. Marmain et al. (2014) assimilated radial velocity observations from the Toulon HFR system in a regional model in the Gulf of Lion. They showed how HFR observations can be successfully used to correct the wind forcing used to constrain the model coastal surface circulation. In the Ligurian Sea, Vandenbulcke et al. (2017) were able to correct surface currents and improve the representation of inertial oscillations after the assimilation of all the available hourly radial observations in a regional model of the area. Variational methods were also applied to improve model dynamics through multi-platform data assimilation including HFR in the southern Tyrrhenian Sea (Iermano et al., 2016) and in the Adriatic Sea (Janeković et al., 2020).
More recently, Hernández-Lasheras et al. (2021) specifically assessed the impact of assimilating HFR observations on the surface currents in the Ibiza Channel using the WMOP operational system. They compared the performance of both radial and total daily mean HFR-Ibiza surface currents (Tintoré et al., 2013, 2020) for correcting mesoscale and sub-mesoscale circulation using different initialization methods in an operational-like context. An independent Lagrangian validation performed by comparing non-assimilated and assimilated (without and with HFR) measurements with a set of 14 surface drifters (Tintoré et al., 2014) showed that the best results were obtained when using HFR total observations along with the traditional observation sources (i.e., satellite altimetry, sea surface temperature –SST, and Argo temperature and salinity profiles). After 48 h, the mean separation distance between virtual buoys and real drifters was reduced by 53 % compared to the simulation without any data assimilation and by 29 % compared with the simulation assimilating traditional observations only (as shown in Fig. 5).
To the best of the authors' knowledge, SOCIB WMOP (https://www.socib.es/?seccion=modelling&facility=forecast, last access: 9 May 2022) is presently the only system in the Mediterranean Sea including an assimilation scheme of HFR data in its operational chain.
2.1.3 Short-term predictions
Assimilation of HFR data into models is still computationally expensive and a complex issue, not to mention operational capabilities of such a procedure. Because of these constraints, the availability of real-time high-resolution HFR current fields has led to alternative solutions in order to obtain short-term prediction (STP) of surface coastal currents through the direct use of HFR historical and nowcast observations using different approaches (e.g., Zelenke 2005; Frolov et al., 2012; Barrick et al., 2012; Orfila et al., 2015; Vilibić et al, 2016).
The abovementioned studies develop and implement different STP approaches (harmonic analysis of the last hours, genetic algorithms, numerical models, etc.), which often require additional data or long training periods of data without gaps. Hardware failures due to power issues, communications or environmental conditions often result in spatiotemporal gaps within HFR datasets. Spatial gaps can be filled on a real-time basis, but the filling of long temporal gaps is not straightforward. Several gap-filling methodologies have been developed for HFR datasets: open modal analysis – OMA (implemented by Lekien et al., 2004, and further optimized by Kaplan and Lekien, 2007), data-interpolating EOFs – DINEOF (Beckers and Rixen, 2003; Alvera-Azcárate et al., 2005; Hernández-Carrasco et al., 2018b, Bourg and Molcard, 2021), self-organizing maps – SOMs (Kohonen, 1982, 2000, 2001; Hernández-Carrasco et al., 2018b), reduced-order optimal interpolation – ROII (Kaplan et al., 1997), optimal interpolation – OI (Kim et al., 2008), artificial neural network – ANN (Ren et al., 2018), variational analysis (Yaremchuk and Sentchev, 2011) and data-interpolating variational analysis in n dimensions – DIVAnd (Barth et al., 2021). HFR-derived short-term predictions were developed by Zelenke (2005), Frolov et al. (2012), Barrick et al. (2012), Orfila et al. (2015), Solabarrieta et al. (2016), Vilibić et al, (2016) and Abascal et al. (2017). More recently, Solabarrieta et al. (2021) developed a Lagrangian-based, empirical, real-time, short-term prediction (L-STP) system in order to provide short-term forecasts of up to 48 h of ocean currents from HFR data.
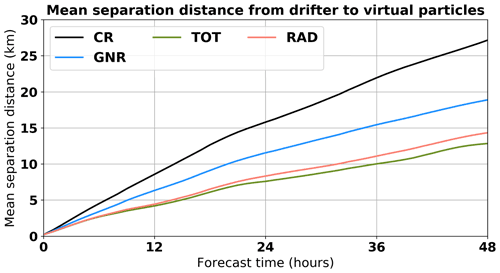
Figure 5Mean separation distance between virtual particles and drifters as a function of the forecast horizon. Black represents the simulation without DA (i.e., control run, CR). Blue stands for generic (GNR), which assimilates data from satellite altimetry, SST and Argo profiles. Green and red lines represent the simulation which assimilates HFR daily mean total (TOT) and radial (RAD) observations, respectively, together with generic observation sources. Original source: Hernández-Lasheras et al. (2021).
Through the NEURAL project (http://www.izor.hr/neural, last access: 9 May 2022), an innovative neural-network-based ocean forecasting system has been developed, providing gridded hourly surface current forecasts in the northernmost part of the Adriatic for the next 72 h. The forecasting system uses an unsupervised neural network algorithm, called self-organizing maps (SOMs, Kohonen, 1982; Liu et al., 2006), to train joint solutions coming from HFR measurements and a numerical weather prediction model as hourly surface currents and surface winds, respectively. Once the joint SOM solution has been trained, the surface current forecast follows the predicted surface winds that are the closest to the specific SOM solution (Fig. 6). Such a system requires a strong relationship between the predictor (i.e., surface winds) and the predictand (i.e., surface currents), which is largely found in coastal regions of the Mediterranean, yet it can be applied for any other combination of predictors and predictands. Also, high-frequency processes such as tides are removed from the system as being minor to the wind-driven dynamics, yet the tides can be added to the forecast.
The quoted northern Adriatic forecast system has been trained using 20 SOM solutions (so-called Best Matching Units, Liu et al., 2006) on HFR data measured between February and November 2008 conjoined with 3-hourly surface winds interpolated to 1 h resolution coming from the Aladin/HR operational model run once a day by the Croatian Meteorological and Hydrological Service (Tudor et al., 2013). The forecasting system performance was tested in the forecast (hindcast) mode during 2009 and 2010. Unfortunately, the HFR system has had substantial problems since 2010 and the antennas were eventually removed in the following years, resulting in a relatively short dataset that is possibly not sufficient for strong reliability of the forecasting system solutions. However, Vilibić et al. (2016) compared this SOM-based surface current forecast system (available online in http://jadran.izor.hr/neural/index.htm, last access: 9 May 2022) with the operational ROMS – Regional Ocean Modelling System (Shñhepetkin and McWilliams 2003, 2005) for the Adriatic, with the former lower showing biases and root mean square errors.
2.2 Extreme hazard coastal monitoring
Under the current climate-change scenario, no portion of the coastline is safe from the threat of met-ocean hazards, which are expected to increase in frequency, duration and virulence during the 21st century (Mitchell et al., 2006; Stott, 2016). HFR constitutes a profitable asset for wise decision-making since it presents a wide range of practical applications, including the effective monitoring in near-real time of extreme coastal hazards such as (i) extreme wind events, (ii) severe river discharges, (iii) record-breaking storms and (iv) strong flow reversals (all abovementioned events are addressed in Sect. 2.2.1.), (v) storm surges, (vi) tsunamis (in Sect. 2.2.2), and (vii) typhoons and hurricanes (Barrick and Lipa, 1986; Miles et al., 2017; Lipa et al., 2019).
In response to the increasingly frequent extreme events associated with climate change, their detailed characterization by means of surface current, wave and wind maps derived from HFRs may aid blue economy development in coastal vulnerable areas of the Mediterranean region. Regardless of this, the increasing retrieval of wave and wind maps derived from HFRs (Lorente et al., 2022) is very relevant for the development of renewable ocean energy, an emerging and innovative blue economy sector.
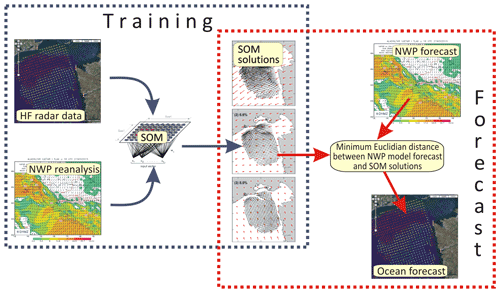
Figure 6The architecture of the SOM-based surface current forecasting system in the northern Adriatic. Original source: from Vilibić et al. (2016).
2.2.1 Extreme event monitoring
HFRs were used to investigate the upper ocean response to an extreme wind event in the Ligurian Sea (NW Mediterranean) during October–November 2018, as described in Berta et al. (2020). This work focused on the analysis of coastal sub-mesoscale structures, shaping surface currents and passive transport. The authors estimate the pattern and magnitude of kinematic properties (e.g., divergence and convergence as well as vorticity patterns) derived from surface currents measured by the HFR-TirLig network (Fig. 1) to characterize the evolution (before and after the event) of ocean scales at a few kilometers.
During the storm, sea surface vorticity (Fig. 7, top panels) and divergence (not shown but available in Berta et al., 2020) reach the order of the Coriolis parameter f, indicating ageostrophic activity typical of sub-mesoscale structures. The evolution of the sea surface structures suggested nonlinear interactions with the wind forcing. Considering the time series of wind speed and sea current properties (Fig. 7, lower panels) during and right after the storm (around 29 October), current magnitude increased approximately 4 times, while vorticity and divergence associated with the small features almost doubled. Such abrupt changes in horizontal currents and transport might also impact the vertical properties and in turn the ecosystem.
In the delta of the Ebro River (NW Mediterranean), the HFR-Ebro system observations have been crucial to capture the evolution of the most extreme Ebro River freshwater discharge event registered over the last 15 years in April 2018 (Ruiz et al., 2020). Results show the high impact of the freshwater pulse discharged on the surface circulation pattern, exhibiting a clear correspondence to high concentrations of satellite-derived chlorophyll a (Chl a hereinafter) concentration. Hovmöller diagrams of HFR-derived meridional and zonal currents indicate an increase in the southeastward velocity during the period of extreme river discharge. The proper representation of the basic oceanographic features of the HFR-Ebro, as the Ebro River impulsive-type freshwater discharged, was previously reported by Lorente et al. (2015).
This same region (i.e., Ebro Delta) was severely impacted by an exceptional storm in (19–24) January 2020, which surpassed the 99th percentile for several parameters (i.e., wind speed, significant wave height, wave period and surface current velocity) compared with the climatology and with a previous storm in January 2017. Particularly for this event, Lorente et al. (2021) have assessed the ability of the HFR-Ebro to characterize waves and currents under the record-breaking storm Gloria. By analyzing the data from the HFR-Ibiza and HFR-Gibraltar, the authors have also evidenced Gloria's remote effect in the Ibiza Channel and the Strait of Gibraltar, altering the usual water exchanges between adjacent sub-basins. Furthermore, the effect of Gloria was also manifested in the highest rates of particle dispersion at the Ebro River mouth on 21 January.
As in the previous case, the HFR system strategically installed in the Strait of Gibraltar (SoG) is considered an appropriate asset to effectively monitor the Atlantic Jet (AJ) inflow (Lorente et al., 2019b) and the water exchanges between the Atlantic Ocean and the Mediterranean Sea. The classical picture of the surface circulation is characterized by current pulses often exceeding 2 ms−1 and time-averaged northeastward speeds around 1 ms−1 in the narrowest section of the SoG (Fig. 4a). Complete collapse of the AJ and quasi-permanent inversion of the surface inflow during prevalent intense easterlies is a singular phenomenon that deserved detailed exploration (as previously mentioned in Sect. 2.1.2). Under this temporal premise, a monthly Hovmöller diagram was computed for HFR-derived zonal currents at the selected transect to easily detect a 2 d full reversal episode during March 2017, represented by black boxes in Fig. 8a. The event detected consisted of an abrupt interruption of the eastward inflow and complete reversal of the surface stream through the narrowest section of the SoG (Fig. 8b). The circulation in the easternmost region of the study domain was accelerated up to 0.8 ms−1, following clockwise rotation that likely fed the Western Alboran Gyre (WAG), which was out of the picture.
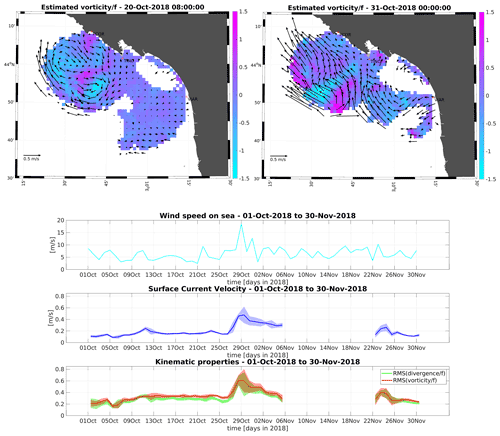
Figure 7From top to bottom: example map of normalized vorticity before and during the extreme event. Time series of wind speed, surface current magnitude, and root mean square of normalized vorticity and divergence. Original source: from Berta et al. (2020).
The prevailing atmospheric synoptic conditions were inferred from ECMWF predictions of sea level pressure and zonal wind at 10 m height (U10), as shown in Fig. 8c–d. A significant latitudinal gradient of sea level pressure was observed, with high pressures over the Gulf of Biscay and isobars closely spaced in the SoG, leading to extremely intense easterlies (above 10 ms−1) channeled through the strait due to its specific geometric configuration. Therefore, high pressures and intense, permanent and spatially uniform easterlies prevailed over the entire study domain, inducing westward outflow through the SoG as revealed by the 2 d averaged HFR circulation maps. Local wind forcing at this scale seemed to play a primary role in explaining such AJ collapse and the related inflow reversals, in agreement with previous studies (Garret, 1983; García-Lafuente et al., 2002; Menemenlis et al., 2007; Péliz et al., 2009; Reyes et al., 2015; Lorente et al., 2019b, Bolado-Penagos et al., 2021).
2.2.2 Tsunami detection
Tsunami early warning and alert represent an emerging and promising application of HFR. The main principle underlying the detection is that the abnormal surface current pattern induced by the orbital velocity of the tsunami wave train can be measured and interpreted in real time by an appropriate detection algorithm. The idea was first proposed by Barrick (1979), but it was only after the 2004 Indian Ocean disaster that the proof of concept was made on the basis of actual HFR data. It was shown numerically with simulated (e.g., Lipa et al., 2006; Gurgel et al., 2011) and real (e.g., Lipa et al., 2011, 2012; Dzvonkovskaya et al., 2012) events that the tsunami signature could be clearly seen in the HFR radial currents, and some appropriate detection algorithms were proposed. One strong point of HFR tsunami detection is that it is not bound to the nature of the source (i.e., seismic or atmospheric) and can be used as a useful complement to other warning systems in places where those are either not available or non-effective. Today, more than 20 real tsunamis have been detected “offline” with the reanalysis of HFR data. In view of the growing interest in these new capabilities of HFR, some radar manufacturers now provide commercial toolboxes along with their hardware system for the early detection of tsunamis; such systems have been installed in some places at risk (e.g., Vancouver Island, Canada; Oman; New Jersey, USA; Sagres in SW Portugal). To date, the only real-time detection was issued following a meteotsunami that occurred on 1 October 2016 in Tofino, BC, Canada (Dzvonkovskaya et al., 2017; Guérin et al., 2018).
However, no such HFR tsunami alert system has yet been installed in the Mediterranean Sea, even though there is a non-negligible tsunami hazard in this region, as witnessed by very destructive co-seismic events in recent history (e.g., Messina, Sicily, 1908). Some worst-case scenarios with a strong (M7.8) earthquake on the northern Algerian margin predict important tsunami waves with 3–4 m amplitude on the French–Italian Riviera (BRGM, 2007). Moderate earthquakes such as the M6.9 21 May 2003 Boumerdes–Zemmouri are sufficient to cause 1–3 m amplitude harbor oscillations within 40–60 min in the Balearic Islands, which would be the most impacted spot by seismic sources in northern Algeria (Wang et al., 2005; Sahal et al., 2009). The impact on the French Mediterranean coast impacted by a seismic source on the northern Algerian margin is shown in Fig. 9.
In addition to co-seismic tsunamis, frequent meteotsunamis (i.e., tsunamis of meteorological origin) have been reported in the Balearic Islands, named – “rissaga” (Jansa, 2007), Adriatic Sea – “šćiga” (Vilibić and Šepić 2009; Orlić, 2015), Sicily Channel – “marrobbio” (Candela et al., 1999; Zemunik et al., 2021), Malta – milgℏuba” (Drago, 2008), northern Persian Gulf (Kazeminezhad et al., 2021), Black Sea (Vilibić et al., 2021) and Aegean Sea (Papadopoulos, 1993). Even though these events have limited regional impact, they can cause severe local damage in harbors and bays due particularly to the micro-tidal regime, resulting in rapid sea level changes (Vilibić et al., 2021). Indeed, the strongest known meteotsunami in the Mediterranean Sea (and likely in the world) was in the Adriatic, the so-called Great Vela Luka Flood (June 1978), with an amplitude of 6 m and periods of about 20 min, as detailed by Vučetić et al. (2009), closely followed by the event from Ciutadella Harbor (Menorca island, Spain) in June 2006 (Jansa et al., 2007) with 4–5 m of amplitude. Meteotsunamis are caused by atmospheric disturbances combined with several further possible amplification mechanisms of the induced sea surface wave; these are mostly the so-called Proudman, Greenspan and shelf resonances in coastal areas, which can lead to strong harbor resonances in semi-closed basins (Orfila et al., 2011). Today, the generation of these meteotsunamis is better understood (Monserrat et al., 2006; Šepić et al., 2009, 2015; Vilibić and Šepić 2009; Ličer et al., 2017), but their prediction is still a very challenging task (Denamiel et al., 2019, Romero et al., 2019, Mourre et al., 2020).
When located in the areas affected by meteotsunamis, HFR-based tsunami early warning systems could be a useful complement to these forecasting systems, helping to issue specific alerts on the basis of the actual observed surface currents 20–40 km offshore a few minutes before the generation of extreme sea level oscillations. Note that tsunami early warning systems only require software updates of existing HFR and could be installed at reduced cost in some places. However, some strategic spots are not covered yet and would need a novel installation to monitor the travel directions of incoming waves from the most probable sources (northern Algerian earthquake, western Corsica submarine mass failure, northern Ligurian earthquake, etc.). Another related issue is extending the range of these HFRs, which would imply operating at lower-frequency bands (4–5 or 9 MHz) than those usually employed in the Mediterranean region (13, 16 or 25 MHz). An HFR prototype (i.e., Stradivarius radar) operating at 4.5 MHz with 200–300 km range was developed by Diginext Ltd. a few years ago for the Gulf of Lion as a proof of concept (Grilli et al., 2015). Such HFR systems can serve the double purpose of warning and characterizing abnormal surface current patterns arising from tsunami-like waves of seismic or atmospheric origin. As recently suggested by Domps et al. (2020), they can also be used as proxies for the observation of low-pressure fronts of atmospheric gravity waves that could lead to storm surge, if not meteotsunamis.
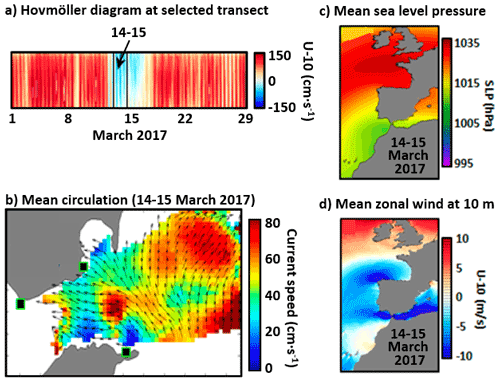
Figure 8(a) Monthly Hovmöller diagram of HFR-derived zonal current speed at the selected transect (shown in Fig. 4a). Red (blue) represents eastward (westward) surface flow. A 2 d episode of permanent flow reversal is marked (14–15 March). (b) HFR-derived mean surface circulation for 14–15 March 2017: permanent flow reversal. (c–d) 2 d mean sea level pressure and zonal wind at 10 m height (u10), respectively, as provided by the ECMWF: intense and persistent easterlies were the driver of the flow reversal. Original source: from Lorente et al. (2019b).
2.3 Environmental transport processes
In the Mediterranean, as elsewhere in the world, the coastal zones serve as the main entry point of nutrients, pollutants and sediments into the ocean, with the multi-scale coastal ocean dynamics being the key drivers for their transport, also impacting their dispersal and retention as well as the cross-shelf exchanges. HFRs have demonstrated a capacity to provide very valuable measurements to continuously monitor the mesoscale structures and frontal dynamics that organize the coastal surface flow and associated transport through the developments in the understanding of Lagrangian dynamics from HFR data (Rubio et al., 2020). The comprehension of the coastal ocean conditions and variability underlying ocean productivity that correlate with fish stock abundance, fish recruitment in coastal areas, and dispersion and retention of larvae is critical for sustainable management of fisheries resources (Sciascia et al., 2018). Coastal and littoral areas are also very vulnerable target regions for pollution in terms of environmental and ecosystem impact as well as economic and societal consequences, also being essential to assist water quality management by tracking the source and drift of contaminants (e.g., chemical, sewage, oil spills or harmful algal blooms). In the Mediterranean Sea, the applicability of HFRs in the study of environmental transport processes is particularly relevant due to its limited exchange with the oceanic basins, its microtidal character, and its intense internal mesoscale and sub-mesoscale circulation. These specific characteristics of this semi-enclosed sea reduce the potential of dilution and dispersion of dissolved and particulate waste, maximizing the impact of one of the most commonly identified threats (i.e., marine litter and contaminants). In addition, despite being considered one of the most oligotrophic areas in the world ocean, it is also one of the world's hot spots for biodiversity (Coll et al., 2010, Gabrié, et al., 2012), providing vital areas for the reproduction of pelagic species (e.g., Atlantic bluefin tuna, white shark and sea turtles) and hosting sensitive ecosystems in the shallow coastal waters (e.g., seagrass meadows of the endemic Posidonia oceanica, the key intertidal habitat of vermetid reefs built by endemic gastropod Dendropoma petraeum and coralligenous assemblages, as detailed by Coll et al., 2010).
2.3.1 Pollution and floatable tracking
Coastal regions in the Mediterranean Sea are heavily inhabited, with strong tourism pressure and maritime activity resulting in human and industrial waste intentionally or accidentally dumped into coastal waters. These pollutants evolve according to their chemical transformation over time, are caught in the 3D general circulation, and are carried offshore and to other distant coastal areas by currents. For example, heavy metals or other chemical contaminants that may be present in semi-enclosed harbors (Tessier et al., 2011) could have important consequences for the ecosystem, and plastic litter is currently a massive and particularly harmful component (e.g., Ryan et al., 2009; Declerck et al., 2019) of the marine pollution in the Mediterranean Sea.
Furthermore, detecting, monitoring and cleaning up oil slicks following an offshore spill before they reach the coast is a major challenge. Consequently, monitoring, understanding and forecasting coastal dynamics are critical steps to develop adequate strategies to mitigate the effects of pollution in marine environments from or towards coastal areas. However, forecasting coastal dynamics is one of the most challenging issues in geosciences due to their strong space–time variability as well as the complexity of the processes controlling the dynamics that interact simultaneously over a broad range of time–space scales, as previously highlighted in Sect. 2.1.2. Thanks to the growing importance of HFR as a key element of coastal observing systems, coastal currents can nowadays be continuously measured in relatively large coastal areas, thus enabling the analysis of transport properties of the surface flow by means of different diagnostics based on the Lagrangian approach (i.e., addressing the effects of the spatiotemporal variability of the velocity field on drifted particles or tracers). Recent studies have demonstrated the potential of this land-based remote sensing technology for different applications in the field of tracking oil spills (Abascal et al., 2009), marine litter (Declerck et al., 2019) or phytoplankton (Hernández-Carrasco et al., 2018a).
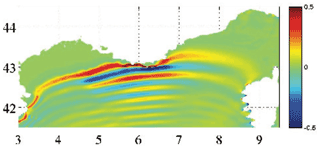
Figure 9Map of the French Mediterranean coast (close to the HFR-Tln system) within the simulated surface elevation (in meters) after 1 h 10 min propagation for a tsunami generated by a M7.8 seismic source on the northern Algerian margin. The numerical simulation uses FUNWAVE-TVD software with three nested grids in the western Mediterranean basin (courtesy of Stephan Grilli, University of Rhode Island, USA).
In this regard, the trajectories of passive tracers are determined by the velocities measured by HFR (e.g., Ullman et al., 2006), often including the effect of subgrid-scale diffusion. When chemical pollutants are considered, additional processes should be included. For instance, when simulating oil spill trajectories, the advection term is a linear combination of the surface current velocity, the wind velocity and the stokes drift, and spreading, evaporation and emulsification should be included in the transport model.
Here we provide evidence to support the reliability of HFR currents for tracking substances in coastal areas. The Lagrangian validation has been performed using data from eight drifter trajectories available in the domain of the HFR area of coverage in the Ibiza Channel (HFR operated by SOCIB, Tintoré et al., 2013, 2020) during October 2012. We use the HFR velocity fields to compute the Lagrangian coherent structures (LCSs), which are very suitable to provide a template of the fluid flow transport (see Haller, 2015, and references therein), allowing the detection of transport barriers, which are of great relevance for marine dynamics. For example, LCS obtained from ridges of the finite-size Lyapunov exponents have been correlated with filaments of remotely sensed Chl a (Lehahn et al., 2007; Hernández-Carrasco et al., 2014, 2018a, 2020), sea bird foraging behavior (Tew Kai et al., 2009), the modeled extension of oxygen minimum zones (Bettencourt et al., 2015) and wind forcings (Berta et al., 2014b). At coastal scales, the dynamical picture in the Lagrangian frame has been analyzed using data from HFR currents to identify relevant small-scale transport barriers (Lekien et al., 2005; Gildor et al., 2009; Rubio et al., 2018), some of them focusing on coastal areas of the Mediterranean Sea (Haza et al., 2010; Berta et al., 2014b; Hernández-Carrasco et al., 2018a). Figure 10 shows the evolution of a set of virtual neutrally buoyant particles initially deployed on the northern (blue dots) and southern (black dots) flank of a given LCS measured from the HFR-Ibiza on 25 January at 17:00 UTC in 2013 (Fig. 10a). Although the location and magnitude of this LCS evolve in time, the LCS persists for several hours, manifesting the presence of a coherent transport barrier preventing the two sets of particles from being mixed up. A meridional LCS is formed and maintained during the simulated period, limiting water exchanges between the coast and the open ocean (Fig. 10a–c).
Another example of application of the Lagrangian properties derived from HFR focuses on the monitoring of the physical mechanisms that can influence the escape times of mesotrophic and/or polluted coastal waters to oligotrophic offshore areas, as described in Rubio et al. (2020). Using as input gap-filled HFR velocity fields a Lagrangian particle-tracking model provides the particle trajectories. From the Lagrangian model outputs, it is possible to infer the characteristic timescales for transport processes in the HFR footprint area by means of the escape rate of active particles (Fig. 11). Thus, HFR is shown to be an excellent tool to monitor conditions and identify the different scenarios that favor the local retention and dispersal of shelf waters in two study areas under the influence of ocean boundary currents.
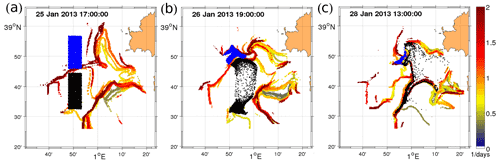
Figure 10Evolution of two sets of particles (black and blue) in the area covered by the HFR-Ibiza in January 2013 superimposed on the backward finite-size Lyapunov exponent – FSLE (color bar). The virtual particles are initially deployed at both sides of a barrier revealed by a zonal LCS on 25 January 2013 at 17:00 evolving for 68 h.
2.3.2 Eddy tracking
Ocean eddies are ubiquitous, pervasive flow structures which dominate the ocean velocity field at several scales from the mesoscale to the local scale (Chelton et al., 2011). They play a fundamental role in sea dynamics, being responsible for the energy transfer among different scales (down to the dissipative range) as well as for their ability to transport nutrients, biomass, sediments and pollutants. Mesoscale eddies, produced by geostrophic instabilities, are not able to advance the energy transfer, being constrained by geostrophic and hydrostatic balance (Charney, 1971). When the balance is broken, the downscale may continue through inertia–gravity waves emitted from currents, ageostrophic instabilities and bottom boundary layer turbulence, which are responsible for the formation of sub-mesoscale eddies. At a lower scale, three-dimensional turbulence proceeds toward the dissipative range (McWilliams, 2019).
The presence of ocean eddies has become more evident in recent years thanks to the introduction of new oceanographic measurement techniques, while their exhaustive characterization would require synoptic time series of the velocity field in the ocean (Robinson, 1983). Such synoptic observations are made available only through satellite data. However, besides being limited to relatively large scales and preferably to the open ocean, they do not provide direct measurements of the total velocity field. Indeed, altimetry data can be used to retrieve the surface geostrophic field, which lacks a possibly important portion of the dynamics (Rinaldi et al., 2010; Conti et al., 2016). Other ways to observe eddies from satellites consist of the observation of their presence in the sea surface temperature or in the tracer field patterns, as displayed by ocean color (Robinson, 2010). Coastal HFRs overcome all the above limitations, enabling the detection and tracking of the time history of surface eddies down to sub-mesoscale at the cost of a reduced spatial extent.
Mandal et al. (2019) provide quite an extensive list of recent literature reporting sub-mesoscale features observed by HFRs, with examples of observations in various coastal areas from the Atlantic to the Pacific and Indian oceans (Shay et al., 1995, 2000; Kirincich, 2016b; Archer et al., 2017; Lai et al., 2017; Arunraj et al., 2018). It is worth noticing that such features may have a strong vertical signature that HFR data fail to account for and therefore need to be complemented by further information spanning from direct measurements of the horizontal and vertical velocity profile to indications indirectly derived from, e.g., satellite turbidity measurements (see discussion in Uttieri et al., 2011).
A vast HFR network now covers the northwestern Mediterranean coastal areas (see Lorente et al., 2022), which is characterized by significant mesoscale variability and eddy generation that, in some cases, shows recursive and seasonal patterns. Allou et al. (2010) used HFR to observe and characterize vortex structures, mostly anticyclonic, in the Gulf of Lion. They also argued they were correlated with specific wind patterns. Schaeffer et al. (2011) improved the analysis and employed both HFR measurements and numerical modeling to analyze the eddy-generating mechanism. They found it is primarily influenced by wind forcing and its interaction with topographic constraints (northerly offshore wind), as well as freshwater input from the Rhone river (southerly onshore wind). The combination of HFR, in situ observations and modeling tools allowed Guihou et al. (2013) to identify an anticyclonic coastal eddy which was generated in front of Nice by a meander of the Northern Current and advected downstream toward the Toulon area, interacting with the mean circulation. More recently, the analysis of the long 2012–2019 HFR time series in Toulon allowed identification of cyclonic and anticyclonic recurrent eddies mainly generated by wind and boundary current undulations (Bourg and Molcard, 2021).
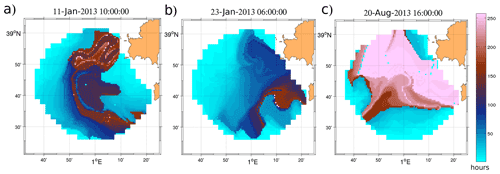
Figure 11Maps of particle residence times (hours) computed for different dates and seasons from HFR observations in the Ibiza Channel SOCIB (a, b for winter and c for summer conditions). Original source: from Rubio et al. (2020).
The development of monitoring networks providing long time series of data is making automatic eddy detection methods more and more topical and important. Generally speaking, existing eddy detection algorithms can be divided into three families: (i) those that are based on the geometrical features of the velocity field, typically in terms of streamline closedness, winding angle or vector geometry (Sadarjoen et al. 1998; Heiberg et al., 2003; Ebling and Scheuermann 2003; Nencioli et al., 2010); (ii) those based on dynamical characteristics, such as parameters quantifying the eddy intensity and its vorticity (Jeong and Hussain 1995; Fang and Morrow 2003; Isern-Fontanet et al., 2003; Morrow et al., 2004); (iii) and hybrid methods based on the combination of geometric and dynamical criteria (Mkhinini et al., 2014; Conti et al., 2016, see also the extensive review in the paper by Le Vu et al., 2018).
Algorithms specifically devised for HFR data are very few. The methods tested in the Mediterranean Sea (Caldeira et al., 2012) are limited to those by Nencioli et al. (2010) and Bagaglini et al. (2020). The former, even though developed for HFR data (and for high-resolution numerical model outputs), has found a widespread range of applications to observations collected by different platforms, as witnessed by current oceanographic literature (Liu et al., 2012; Dong et al., 2014). It is a method based on the geometry of the velocity vectors. It was conceived for geostrophic or quasi-geostrophic recirculating features showing very little divergence. For this reason, it is very suitable to describe mesoscale eddies but may fail in detecting sub-mesoscale ones, which often are characterized by divergence or convergence and by a high degree of deformation of the velocity field geometry. YADA (Yet Another eddy Detection Algorithm), developed by Bagaglini et al. (2020), was conceived specifically to overcome this limitation and be utilized to automatically detect sub-mesoscale eddies, which may exhibit highly non-geostrophic characteristics. It is a hybrid method, which focuses on both the dynamical and geometric features of the velocity field, first identifying the local extrema of a dynamical field characterizing recirculations (e.g., the local normalized angular momentum, see Mkhinini et al., 2014; the Okubo–Weiss parameter, Okubo, 1970; Weiss, 1991), similarly to the first step from AMEDA (Angular Momentum Eddy Detection and tracking Algorithm) defined by Le Vu et al. (2017), and thereafter analyzing the streamline geometry in a neighborhood of the extremum. YADA (Bagaglini et al., 2020) has been successfully applied to 1 km resolution HFR data from the Gulf of Naples, showing its ability to identify strongly asymmetric, convergent or divergent sub-mesoscale eddies. Its application to coastal HFR data from other areas of the western Mediterranean is presently under way.
The HFR system from the LaMMA Consortium (described in Lorente et al., 2022) covers part of the Ligurian Sea and the Tuscany Archipelago, which is a shallow area separating the Ligurian and Tyrrhenian basins, bordering the Corsica Channel eastward, with complex topography and coastal morphology, also due to the presence of several islands (Elba, Capraia, Montecristo, Gorgona). Sea dynamics are strongly influenced by seasonality and characterized by the presence of the Tyrrhenian boundary current and its bifurcation, the Eastern Corsica Current (Astraldi and Gasparini, 1992; Millot, 1999; Vignudelli et al., 2000). Through drifters, in situ data and a numerical model, Poulain et al. (2020) studied the area in the summer season. They found prevailing southward current flowing next to the Italian coast, then turning westward and northward, encountering Elba island. A further eastward motion led to the formation of an anticyclone centered on Capraia island, which exhibited variations correlated with wind forcing. The presence of an anticyclone north of the Corsica Channel in summer and autumn seasons was previously documented by Ciuffardi et al. (2016) by means of in situ profiles and altimetric data. Furthermore, they argued that the characteristics of the anticyclone (position and size) may affect the general circulation by isolating the Tyrrhenian and Ligurian basins, mostly in summer. These hydrodynamics features can influence the concentration of floating marine litter, which was shown to be particularly high in certain periods of the year (Fossi et al., 2017).
Here, we show the application of both YADA and the Nencioli et al. (2010) algorithms to the surface currents derived from the HFR-LaMMA system during the year 2019.
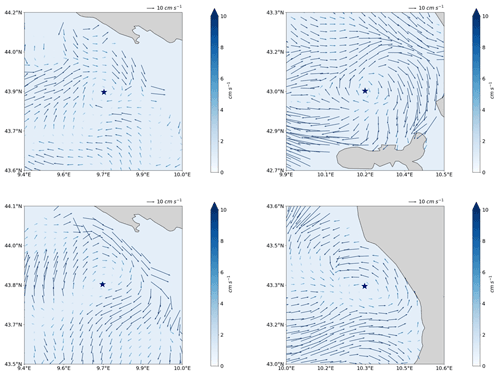
Figure 12Maps of the area of the eastern Ligurian Sea and Tuscany Archipelago showing the HFR-derived surface currents (colored arrows indicate the current speed) and the detection of four eddies in early August 2019 by YADA. The blue star marks the eddy center.
Figure 12 reports four eddies detected by YADA and the corresponding surface currents in August 2019.
Figure 13 shows the results of the application of the algorithm by Nencioli et al. (2010) to the whole year 2019, which provided a seasonal census of anticyclonic and cyclonic eddies in the area sampled by the HFR-LaMMA system. The HFR coverage was not uniform during the year, with generally lower percentages for the warmer seasons (i.e., spring, summer). The area north of Elba island was characterized by the highest eddy activity throughout the year, with a predominance of anticyclonic eddies in colder seasons and a clustered pattern in summer, showing anticyclonic eddies east of Capraia island and cyclonic ones toward the coast.
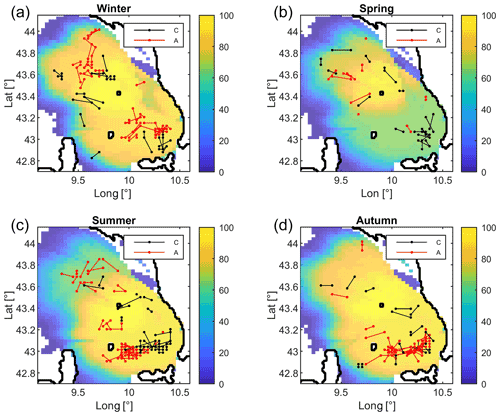
Figure 13Maps of the area covered by the LaMMA HFR network showing the percentage of HFR data availability during 2019 for (a) winter, (b) spring, (c) summer and (d) autumn. The Tuscany Archipelago (i.e., Elba island) is at the lower right corner of the figures. Tracked cyclonic (black) and anticyclonic (red) eddies detected in each season are overlaid.
The number of detected eddies depended also on the availability of HFR data; indeed, a smaller number of eddies was found in spring (28) with respect to the other months (62 winter, 68 summer, 62 autumn). Median eddy life span was around 1.125 d for all seasons except in spring, although some were able to survive up to 6 d.
Although the present work is preliminary, it may lay the foundation for a detailed analysis concerning the seasonal features of eddy activity within the Tuscany Archipelago and its effect on the general circulation. Surface currents from HFR can be combined with numerical model outputs to bridge the gap relative to the spatial and temporal coverage of data and to improve the representation and forecast of the real sea state: HFR observations enhance numerical simulations by resolving fine-scale processes in intricate regions with complex geometry configurations. In turn, hydrodynamic models can reciprocally serve as integrative connectors of sparse in situ observations and gappy HFR surface current maps by offering a seamless predictive picture of the three-dimensional ocean state.
2.3.3 Transport of biological quantities and connectivity
The necessity to preserve the marine ecosystem equilibrium and the water quality has fostered the use of HFR data in supporting coastal zone management and assessing the variability in the dynamics of marine ecosystems. In particular, HFR data have been used worldwide to address ecological and water quality issues such as (i) to understand the transport and retention processes of plankton or wastewater discharge in some regions of NW Spain (Ria de Vigo) (Piedracoba et al., 2016) and the western Mediterranean (Hernández-Carrasco et al, 2018a), at coastal upwelling fronts off central California (Bjorksted and Roughgarden, 1997) and in Monterey Bay (Coulliette et al., 2007); (ii) to investigate the enhancement of productivity due to the retention of phytoplankton within the flow in the Santa Barbara Channel (Brzezinski and Washburn, 2011); and (iii) to analyze the relationship between populations of larval and juvenile fishes and the mesoscale flow field in the California Current System (Nishimoto and Washburn, 2002).
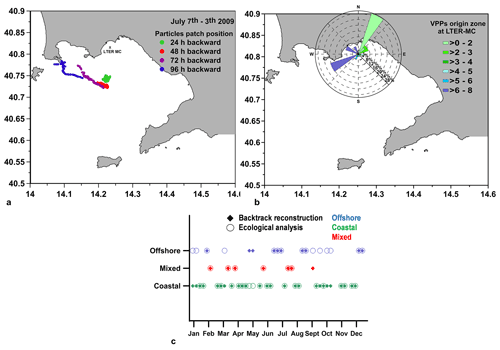
Figure 14(a) Map of the Gulf of Naples (southern Tyrrhenian Sea) showing the (a) Lagrangian backtracking trajectories of virtual phytoplankton patches (VPPs) in the period 3–7 June 2009 and (b) main origin sectors of the VPPs at the LTER-MC site resulting from backward simulations; index sectors of VPPs are 1–2–3–4–5 for coastal areas and 6–7–8 for offshore areas. (c) Comparison among backtracked Lagrangian origin and ecological analysis based on salinity and Chl a data measured weekly at LTER-MC. Green: VPPs originating from coastal areas; blue: VPPs originating from offshore areas; red: VPPs originating partly from coastal and partly from offshore areas. Adapted from Cianelli et al. (2017).
Here we present examples of application of HFR data to investigate ecological questions in three Mediterranean coastal areas: the Gulf of Naples (GoN, Tyrrhenian Sea), the Gulf of Manfredonia (GoM, western Adriatic Sea) and the Malta–Sicily Channel (mid-Mediterranean Sea).
In the GoN, continuous observations of the HFR current fields highlighted several characteristics of the surface circulation and water exchange between the interior of the gulf and the neighboring open Tyrrhenian Sea (Cianelli et al., 2013). An oscillating plankton population dynamic has also been frequently observed in the GoN, at Long-Term Ecological Research station MareChiara (LTER-MC), where plankton abundance has been monitored weekly since 1984. A proof-of-concept study (Cianelli et al., 2017) was thus conducted in order to characterize the spatial scales and the provenance of phytoplankton assemblages detected at LTER-MC and to dissect processes regulating plankton dynamics.
The study focused on a year-long analysis carried out for 2009, which was characterized by a very accurate estimate of the surface dynamics, with a reduced number of gaps among ecological measurements and HFR data. The approach followed these conceptual steps: (i) reconstruction of the annual and seasonal regimes of HFR currents detected at the LTER-MC site and (ii) computation of Lagrangian backtracking simulations advecting virtual phytoplankton patches (VPPs) in the HFR field (Fig. 14a). VPPs were released at the LTER-MC site on the dates of the weekly oceanographic campaigns and tracked backward, thus allowing the estimation of the positions of the VPPs up to 4 d (i.e., 96 h) prior to arrival at LTER-MC. The next step was (iii) identification of the prevailing directions from which the VPPs arrive at the LTER-MC site as resulting from backtracking simulations (Fig. 14b), also allowing the definition of the spatial distribution of the VPP origin zones (not shown) in the GoN. This was followed by (iv) comparison among backtracked Lagrangian reconstruction and ecological analysis based on salinity and Chl a data obtained through weekly sampling at LTER-MC (Fig. 14c) as well as (v) identification of different modes of coupled physical and ecological functioning in the GoN as resulting from physical transport and biological processes (“allogenic” and “autogenic” factors, respectively).
The results showed an alternation in plankton dynamics between phases reflecting the influence of coastal (green) and offshore (blue) circulation patterns on the biological community. The phytoplankton community detected at LTER-MC generally originated from the coast, whereas the offshore inflow marginally changed the main traits of phytoplankton assemblages. Backtracking simulations and biological data strictly agree, highlighting that the plankton community at LTER-MC during 2009 was affected by the alternation of coastal and offshore influence.
Biological autogenic factors drive the modifications of coastal phytoplankton communities during coastal “green” phases, thus suggesting that the GoN tends to retain the same communities via coastward circulation, especially during summer. Physical allogenic factors are important in driving dilution and species advection of coastal phytoplankton during the offshore “blue” phase. This marked alternation between coastal and offshore water masses acts to promote phytoplankton diversity because the dilution in the phytoplankton density may decrease the impact of the dominating species over the available resources.
The integration of long-term biological data and high-resolution current fields represents an optimal tool to investigate the role of surface circulation in structuring the marine plankton community, thus confirming the value of HFR systems to analyze the seasonal fluctuations in marine ecosystems dynamics and to unveil the mechanisms of coastal connectivity.
The GoM is a well-known recruitment area in the Adriatic Sea (Sciascia et al., 2018; Corgnati et al., 2019a). In this region, HFRs have been used to understand the role of ocean currents in the recruitment of small pelagic fishes (i.e., European sardines, Sardina pilchardus). Figure 15 shows residence times within the GoM, estimated using trajectories of virtual drifters computed from the surface currents measured by HFRs. Months with high (October) and low (February) residence times are associated with weaker and stronger surface currents in the central area of the gulf. The relatively short (<12 d) average residence times have shown that local spawning is less likely to take place than transport to the gulf from remote spawning areas through advection pathways. Results agree with otolith measures, suggesting that the arrival of larvae within the gulf is characterized by repeated pulses from remote spawning areas that are likely to play a fundamental role in maintaining the nursery.
The Malta–Sicily Channel is both the most fished area in western Mediterranean Sea and a very important hot spot of biodiversity (Médail and Quézel, 1999). In this region, Capodici et al. (2018) combined the HFR-CALYPSO surface currents together with satellite images of Chl a and SST to explain physical driving mechanisms that can help interpret ocean productivity and plan maritime activities in a more adaptive and proactive way. On the one hand, as mentioned by the authors, monitoring water quality data provides valuable insight into the understanding of processes driving spatial and temporal changes in productivity at sea (Behrenfeld et al., 2006). Chl a and SST are generally accepted as proxies for water quality and are very helpful to detect upwelling events, which frequently occur along the Sicilian coast. In particular, sea currents are responsible for dispersion, transport or retention of nutrients among which Chl a is widely used as a proxy variable; moreover, current jets or eddies are often observable as cold or warm areas in SST maps, respectively. Even if both Chl a and SST maps are usually retrieved by means of satellite data maps, cloudiness often reduces the satellite data availability; thus, temporal aggregated products (e.g., at 8 or 16 d) are the only data available. In this framework, the integration of sea surface current data provided by HFRs can fill the gap of knowledge due to the inadequate temporal (and sometimes spatial) resolution of these water quality maps. Capodici et al. (2018) used principal component analysis – PCA (Preisendorfer, 1988) to firstly extract the dominant spatial patterns of these variables in 2013 (Fig. 16, left panels) and to secondly quantify the degree of correlation between SST (or Chl a) and HFR-derived surface current spatiotemporal patterns (see Fig. 16, bottom right panel). The spatial correlation analysis suggests the importance of current advection in phytoplankton transport, being characterized by fringes where very high positive correlation areas are surrounded by very high negative ones and vice versa.
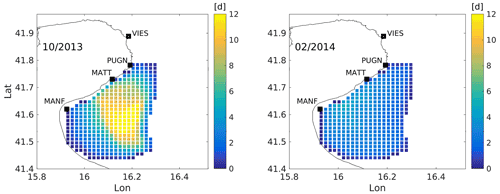
Figure 15Maps of the Gulf of Manfredonia showing monthly bootstrap estimates of average residence times (in days) of virtual particles advected in the HFR velocity field and released within the gulf for the months of October 2013 (left panel) and February 2014 (right panel). Black squares represent the locations of the four HFR antennas along the Gulf of Manfredonia. Adapted from Sciascia et al. (2018).
Moreover, the spatial distributions of time-averaged radar currents and corresponding TKE (total kinetic energy), EKE (eddy kinetic energy), and the absolute value of the products between temporal fluctuations of the deviations from the time-averaged zonal and meridional velocity components (ReS) have shown swirling areas for the first time at high spatiotemporal resolution. The trapping zones, characterized by low values of TKE (see Fig. 16, top right panel), EKE and ReS at their cores, may help explain why this channel is particularly rich in pelagic species, highlighting how continuous high-resolution HFR-derived surface currents can improve decision-making in fisheries resource management.
This initial review of scientific and societal applications using HFR data, implemented in the Mediterranean coastal areas, has allowed us to know their potential and limitations to continue contributing to the regional observing system in addressing the existing regional environmental threats, scientific key priorities and societal needs. Also considering the current threats and the opportunities ahead along the UN Ocean Decade (2021–2030), we have conducted a SWOT (i.e., strengths, weaknesses, opportunities and threats) analysis, as schematized in Fig. 17.
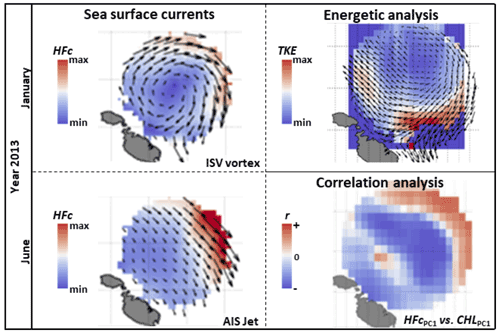
Figure 16Map of the Malta–Sicily Channel showing the monthly averaged HFR sea surface currents for January and June 2013 (top left and bottom left panels, respectively) as well as the spatial distribution of the total kinetic energy (top right panel) and the correlation coefficient between the first PC of both HFR and Chl a (bottom right panel). Original from Capodici et al. (2018), graphical abstract.
One of the main weaknesses found is the limited adoption of common data and metadata models for HFR surface currents. As a result of international and European efforts made in recent years towards HFR data harmonization and distribution, as detailed in Lorente et al. (2022), the following have already been defined: (i) the common data and metadata model for HFR surface currents (Corgnati et al., 2018, 2019b; Mantovani et al., 2020), (ii) the tools for near-real-time (Corgnati et al., 2020) and historical data processing (Corgnati et al., 2019c), (iii) the guidelines (Reyes et al., 2019) and (iv) training activities. Despite that, only 23 % of the near-real-time and 15 % of the historical data from the Mediterranean HFR sites are integrated into the European HFR node (Lorente et al., 2022), which was established in November 2018 as a focal point for data management and distribution.
In the sphere of maritime safety, the main strength of HFRs is the provision of high-spatiotemporal-resolution surface currents over wide coastal areas, where most SAR incidents occur (see Sect. 2.1.1). HFRs are used as alternatives to models for backtracking drifting objects in near-coastal risk-prone environments and for complementing other scarce in situ observations, also helping to assess and to improve ocean models through data assimilation (see Sect. 2.1.2). In this regard, the provision of HFR data uncertainties (Moore et al., 2019) might boost the use of HFR data for data assimilation and model assessment. In addition to that, the machine-learning approach whereby a neural network model is trained on past data and then used to create short-term predictions (see Sect. 2.1.3) has gained ground in recent years. The steady growth of the European HFR network (Rubio et al., 2017; Roarty et al., 2019), increasing both the coastal coverage in many countries and the length of the time series, will allow us to implement these self-learning algorithms in other Mediterranean areas. Nevertheless, there is a strong need for consensus on the methodology to generate these scientific added-value products and on the definition and further adoption of a common data and metadata model as well as quality control tests. This lack of consensus hinders the operational distribution of standardized HFR gap-filled data and derived STPs, which may lock the HFR data potential for their use in several Lagrangian applications.
Additional efforts for unlocking interoperable HFR (basic or added value) data access will greatly contribute to delivering greater uptake, use and value from the HFR data. Once we are able to turn HFR data into customized information, the further challenge is to extend the science-based added-value products and applications to societally relevant downstream services (Tintoré et al., 2019). To face this challenge, a clear stakeholder engagement strategy is needed to identify, categorize and analyze user needs and requirements. Through strengthened links with the identified stakeholders, the HFR community will reinforce the user's loyalty, whereas by involving them along the life cycle of the application development will ensure the achievement of their needs, raising their interest and usage. Furthermore, emerging HFR applications will enable new communities and sectors to discover and use HFR data.
As a land-based remote sensing technology, HFRs are able to continuously monitor the coastal ocean response to extreme events without the need to be deployed at sea under severe met-ocean conditions, thus avoiding the risk faced by other observing platforms (i.e., research vessels, ferry boxes or even autonomous instruments). Moreover, HFRs are also unaffected by cloud coverage, which is usually associated with extreme storms and prevents ocean color satellites and infrared radiometers from inferring and further observing the Chl a concentration and the SST, which are generally used as proxies for surface circulation. In addition, it is worth highlighting that under severe weather phenomena, near-real-time met-ocean information gains value since it is essential to avoid risky situations and to support emergency response at sea. The resilience of HFRs under unfavorable weather conditions has allowed us to monitor and deeply investigate the impacts of intense wind episodes, severe river freshwater discharges and record-breaking storms as well as to observe the weakening or even the reversal of main surface currents and jet streams (Sect. 2.2.1). Furthermore, as it has been demonstrated that tsunami signature, irrespective of its origin (i.e., seismic or atmospheric), can be clearly seen in HFR radial currents, this opens up the possibility of using them as a useful complement to other warning systems in places where those are either not available or non-effective (Sect. 2.2.2). Nevertheless, despite the existing risk from tsunamis and the frequent occurrence of meteotsunamis in particular coastal areas of the Mediterranean Sea, no HFR tsunami alert system has been installed in this region. Facing a growing interest in these HFR new capabilities, several threats must be addressed: (i) the installation of new systems to monitor the most probable source areas and (ii) the extension of the range by using lower operational frequencies in the Mediterranean Sea to be able to detect tsunami-induced currents far offshore to offer early warning.
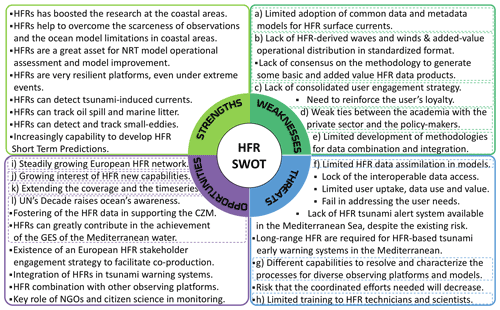
Figure 17SWOT analysis of the HFR capabilities and applications. Dashed-line boxes around the text highlight those weaknesses, threats and opportunities that have been addressed in the recommendations.
The recognized capabilities of continuous HFR observations to analyze the transport properties of the surface flow and to detect and track surface eddies down to sub-mesoscale (Sect. 2.3.2) have allowed us (i) to understand the phytoplankton distribution and to identify different local retention scenarios (Sect. 2.3.1) as well as (ii) to investigate the role played by the characteristic mesoscale variability and eddy generation in the transport of biomass and pollutants as well as in the recruitment and abundance of small pelagic species in the Mediterranean coastal waters (Sect. 2.3.3). These practical applications have fostered the use of HFR data in supporting coastal zone management (CZM) and assessing the variability in the dynamics of marine ecosystems, becoming a valuable asset for contributing to the achievement of Good Environmental Status (GES) of the Mediterranean waters. However, it has been found that the number of detected eddies depends on the HFR data availability, highlighting the need to combine HFR data with numerical model outputs to bridge the spatiotemporal gap of the observed data and to improve the reliability of the simulations. It should also be noted that the limited coverage of HFR reduces the potential of larger-scale applications and connectivity studies, thus requiring their integration with other in situ and satellite observations as well as models, which in turn will benefit from the future expansion of the HFR network.
Considering the key features of HFRs (see Sect. 1) as their main competitive strengths when compared versus other observing platforms, it must be recognized that the provision of information at the very near-surface layer constitutes the most serious limitation, as highlighted in the SWOT analysis from Lorente et al. (2022). In order to fully understand ocean dynamics, knowledge of the 3D processes in the entire water column is essential as well. A significant number of coastal ocean observatories in the Mediterranean Sea (as described by Tintoré et al., 2019) encompass a complex multi-platform network including HFRs, aiming to meet the primary but challenging need to monitor both the surface and the water column. This has motivated the development of techniques able to combine information on the processes in the entire water column in order to provide a three-dimensional picture of the overall dynamics. The combination of such observations is challenging mainly for two reasons: the surface and ocean interior are prone to different processes and forcings with different spatiotemporal scales, and, at the same time, the capabilities to resolve and characterize the diverse processes may be different for observing platforms at the sea surface and in the water column. Despite the promising results obtained by Berta et al. (2018) and Guihou et al. (2013) in the Mediterranean Sea, necessary efforts must continue towards the further development of methodologies to combine HFR data with water column measurements and models.
After the description of the current implementation status of HFR applications in the Mediterranean coastal areas and based on the results obtained from the previous SWOT analysis, we present the prospects for the future and a set of key recommendations. Future prospects for HFR applications will benefit from the progressive implementation of the defined recommendations: (i) to ensure that the potential of HFR data is fully exploited in the development of operational monitoring systems at the regional level; (ii) to help derive the added value achieved by the European HFR network (Rubio et al., 2017, 2021; Corgnati et al., 2021; Lorente et al., 2022), such as data management centralization and standard data distribution, the development of new products and cross-disciplinary emerging applications, and the provision of training workshops; and (iii) to include the recommendations in the long-term monitoring strategy. This last point is crucial for (i) developing and integrating the different COOSs into a robust regional ocean observatory, (ii) ensuring that such integration is fully aligned with the European and global roadmap, and (iii) addressing key science priorities and societal challenges of the Mediterranean coastal regions.
Although the future prospects at the regional level are shared far beyond the geographical borders of the Mediterranean Sea, recommendations have a stronger regional focus: (i) to coordinate cross-national efforts (i.e., implementation of technical, financial and management approaches at national and regional level, establishment of cross-border agreements for obtaining dedicated frequency allocation for HFR technology), (ii) to account for regional specificities (e.g., north–south unbalance in the monitoring capabilities, prominent use of medium- and short-range HFR frequencies, existence of ship noise and radio frequency interferences due to high marine traffic density, high restrictions for obtaining the required HFR installation licenses in coastal tourism areas) and to respond to regional needs (in terms of scientific key priorities, societal needs and existing environmental threats), and (iii) to map the existing and potential HFR data regional end users, also facilitating the interaction with them. On the basis of the future prospects, 12 recommendations can be highlighted in the same alphabetical order as the weaknesses (items a–e) and threats (items f–h) which are intended to be covered, as well as the opportunities (items i–l) to be seized by each one of them (as in the schema in Fig. 17).
- a.
Keep promoting the HFR data interoperability and distribution at the regional level. In order to reduce the bottlenecks that hinder HFR data harmonization and their provision as open data, a data policy or data sharing agreement between the parties providing and receiving data should be defined and put in place. It might also partly resolve the difficulties of sharing the HFR data held by companies or controlled by arrangements with the private sector, who partially or fully funded the installations. The collaboration with international initiatives will ensure convergence and interoperability. The HFR data harmonization and sharing will ultimately contribute to providing the research community with continuous and more valuable coastal data and to underpinning the development of HFR applications.
- b.
Reinforce the Mediterranean's leadership for defining the standard models of HFR basic and added-value products. Mediterranean institutions are key players in the European and international HFR research effort in multidisciplinary fields and in the development of applications, as mostly covered in Sect. 2 of this work. In addition to that, HFR experts from Mediterranean institutions are actively contributing to the definition of the European HFR network roadmap detailed in Rubio et al. (2021), leading crucial tasks. One of these tasks aims to define the standard model and to increase the availability and accuracy of the HFR wave parameters, which are weaknesses included in Lorente et al. (2022). Another important task focuses on seeking consensus on the methodology for the provision of HFR added-value data products (i.e., gap-filling data). The creation of partnerships between different research groups and institutions in the context of European, regional and national projects (Lorente et al., 2022) is contributing to moving forward both the expansion of the HFR network and the main research areas, also fostering HFR data interoperability and distribution, thereby helping to unlock HFR data potential, discovery and usage.
- c.
Enhance data discoverability, access and usability. The EuroGOOS HFR Task Team has already taken the first steps towards the definition of the user engagement strategy (Rubio et al., 2021) of building a database of current and potential stakeholders with the input of some Mediterranean institutions. However, stronger involvement is needed to avoid imbalances between countries and greater efforts are required to move it forward by means of programs that promote networking and coordination. Tight interactions with stakeholders on a fit-for-purpose basis and the enhancement of the societal impact of HFR data are major elements of the strategy, especially towards ensuring long-term sustainability. Boosting the regional involvement in this strategy will result in the spreading of end-user applications at the regional and/or local level, also stimulating the development of new ones in response to user feedback.
- d.
Strengthen public–private partnerships (i) at the regional level by enhancing inter-institutional collaboration to exchange expertise, to build consortia and to share job opportunities in this field. In this sense, the Mediterranean HFR network is benefitting from the activities carried out in the context of the EuroGOOS HFR Task Team, summarized in Rubio et al. (2021), and from ongoing regional joint projects and existing national HFR coordination structures, as listed in Lorente et al. (2022). It is also highly recommended to participate in annual fora led by stakeholders, like the first MED-FORUM that brought together the heads of maritime services or coast guards of almost all Mediterranean states, aiming to develop a regional policy in the field of maritime safety and to improve the efficiency of SAR services in the Mediterranean area (Trevisanut et al., 2010). (ii) At the European level, partnerships could be strengthened by being aligned with ongoing initiatives and projects, thereby contributing to the EuroGOOS HFR Task Team and the main marine data portals (e.g., Copernicus Marine Service, EMODnet, SeaDataNet), which will avoid duplication of efforts. (iii) At the global level, this could be achieved by collaborating with international institutions that are world leaders in HFR and ocean observation as well as with the global HFR network to ensure consistency in data standards and best practices. (iv) With the private sector, partnerships could be strengthened by transferring knowledge and applications from academia to the operational oceanography industry to turn them into commercial services, improving the links between research and new technologies. The development of existing and potential applications will also assist HFR manufacturers in marketing their technologies.
- e.
Foster HFR data integration with other in situ and satellite data. Exploiting the nature of their measurements, HFRs are currently being used for filling the gaps in other sparse or lower-spatiotemporal-resolution observations in coastal areas, as well as for improving and assessing satellite observations. In this context, the opportunity offered by the launch of the wide-swath Surface Water and Ocean Topography (SWOT) altimeter expected in November 2022 should be considered. The SWOT altimeter should be complemented with other remote and in situ sensors to fully resolve the typical Mediterranean mesoscale structures of 10–100 km (Gómez-Navarro et al., 2018). Additional complementarities might be fostered with the monitoring of surface currents worldwide using information from the Automatic Identification System (AIS) data streams (Benaïchoucheet al., 2021), from which HFR measurements can be used as a consistent ground-truth dataset for validation purposes and for increasing the spatial resolution of the AIS-reconstructed fields at coastal areas. The integration of HFR measurements with other multi-platform observations from gliders or acoustic Doppler current profilers (ADCPs) (Manso-Narvarte et al., 2020) has already been implemented and tested, particularly under the umbrella of the JERICO-NEXT project (Griffa et al., 2019). This multi-platform combination underpins a sound understanding of the three-dimensional coastal circulation, allowing the broadening of HFR applications (i.e., below the surface). Data fusion and integration will contribute to increasing the societal and scientific value of all observations, not only the HFR ones.
- f.
Boost HFR data assimilation for model improvement. As shown in Sect. 2.1.2 of the present work, HFR surface current data assimilation has been demonstrated to improve the model performance in many studies (Paduan and Shulman, 2004; Barth et al., 2008; Hernández-Lasheras et al., 2021). Furthermore, HFR standard data distribution in near-real time throughout the main European marine data portals, facilitating data access and ensuring timeliness, makes them ideal for efficient data assimilation in operational modeling (Capet et al., 2020). However, WMOP is the only regional model from the Mediterranean which systematically assimilates HFR data in its operational chain (Hernández-Lasheras et al., 2021). As highlighted by Capet et al. (2020), the lack of expertise, training and capacity building is limiting the uptake of assimilation practices.
- g.
Expand the pool of expertise by including not only HFR technology, but also data management and applications, satellite remote sensing, ocean modeling, data assimilation, training aspects, and exchanging and sharing knowledge, tools, data and know-how between diverse research groups at the European, regional and global levels.
- h.
Train the new generations of HFR technicians and scientists. They are needed to ensure knowledge exchange and sufficient expertise to allow for a significant expansion of the coverage and sustainability of operations and HFR applications. This is currently being done in the context of periodic workshops and summer schools (such as the recent ISSOR and SICOMAR plus summer schools) within academia. Moreover, the development of best practices for demonstrations is not only key to reach satisfactory quality standards but also for fostering the learning process. However, additional technical training courses provided by manufacturers at the HFR operator level are recommended. Furthermore, the participation of Mediterranean institutions and companies in the creation of an international, intersectoral and interdisciplinary qualified supporting training network will contribute to boosting knowledge and know-how exchanges involving all actors (e.g., academia, operators, manufacturers, private sector).
In addition to all abovementioned recommendations, seeking funding is a compulsory task aiming to support, together with stakeholder engagement and the training of new generations, the long-term sustainability of the HFR network, data and applications at national, regional and European levels. To this end, the EuroGOOS HFR Task Team has taken early steps to prepare a competence matrix that will facilitate the building of effective, interdisciplinary, intersectoral and well-balanced consortia, grounded in shared research interests and goals, aiming to prepare competitive bids and applications for funding, taking advantage of the expertise of the team in diverse grant calls (Rubio et al., 2021). In addition to research and grant funding, long-term infrastructure funding at national, regional and European government level with financial input from other operational users through regional consortia will be needed for a truly sustainable infrastructure. For further details about the diverse socioeconomic and technical challenges to be tackled during the implementation of a sustained and integrated HFR regional network, the reader is referred to Sect. 5.1 of the companion publication from Lorente et al. (2022).
The Mediterranean HFR network must also take advantage of the opportunities that arise in the framework of the UN Decade of Ocean Science for Sustainable Development and the European Green Deal to lead the way towards future prospects. The four recommendations given to benefit from the opportunities, as included in the SWOT analysis (Fig. 17, bottom left panel), are as follows.
- i.
Expand the Mediterranean HFR network. Although this network already represents 55 % of the HFR sites existing in the European inventory (Lorente et al., 2022) and despite the very recent installations (i.e., in the port of Licata as well as in Portofino and Celle Ligure – Italy, in the port of Menton and in Mimizan – France, and in Ta' Cenc in Gozo – Malta), with several new deployments in an initial planning stage (i.e., in Haifa Bay – Israel, Sardinia and Sicily islands, Gulf of Genoa, Gulf of Naples, Tuscany Archipelago – Italy, Gulf of Trieste – Slovenia, and in the Aegean – Greece), the Mediterranean coastal areas are still under-sampled. In this regard, it should be noted that the spatiotemporal scales currently provided by the HFRs in the Mediterranean allow us to monitor the current environmental threats adequately but always in limited coastal areas, thus reducing the potential of larger-scale applications (e.g., transport of organic matter and pollutants, connectivity studies, data assimilation into models). Therefore, aiming to improve the strategy of ocean observatories to respond to regional needs for a better understanding of region-specific processes towards a fit-for-purpose design, increased monitoring effort by expanding and improving the HFR network is required, allowing for coverage of a large geographical area on a routine basis. Accordingly, a review of major scientific and social questions is needed including environmental stressors and their impacts in the Mediterranean waters and blue economy sectors to identify the benefit of the new deployments in coordination with current monitoring actions (e.g., to identify gaps for monitoring these risks, ensuring cost-effective observations). To this end, cross-border coordination activities are key for the involvement of other countries bordering the Mediterranean Sea along the eastern and southern coastlines and to address issues related to frequency sharing to avoid interference, as highlighted in Lorente et al. (2022).
- j.
Further develop emerging HFR applications in the Mediterranean. The extension of the HFR surface current to fit multiple purposes, aiming to address single to multiple environmental threats, scientific questions and societal needs, requires a multidisciplinary integrative approach and coordinated monitoring of different essential ocean variables. In this context, it is worth mentioning that HFR multiparameter monitoring of the sea state allows the development of diverse applications to tackle a wide range of coastal threats: (i) monitoring eutrophication in highly productive coastal waters, combining HFR surface currents with thermistor chains, oxygen and turbidity sensors at various depth increments, and addressing physical–biological interactions in coastal basins (Cianelli et al., 2017; Hernández-Carrasco et al., 2018a); (ii) monitoring the transport of floating marine litter and other contaminants using surface current fields from HFRs and models (Declerck et al., 2019); (iii) ship tracking (Dzvonkovskaya et al., 2007; Laws et al., 2016); (iv) early tsunami and meteotsunami detection (Lipa et al., 2006; Monserrat et al., 2006; Guèrin et al., 2008 ; Lipa et al., 2011, 2012; Gurgel et al., 2011, Dzvonkovskaya et al., 2012); (v) freshwater monitoring (Meadows et al., 2013); (vi) extracting new information from HFR signals, aiming to advance the understanding of key processes in coastal areas, such as stratification (Shrira and Forget, 2015), air–sea interaction (Berta et al., 2018) and mixing in the upper ocean, as well as near-surface current shear; and (vii) promoting the HFR use for supporting marine renewable energy resource assessment (i.e., winds, currents, waves) in the coastal zone (Wyatt, 2012, 2021; Basáñez and Pérez-Nuñunzuri, 2021; Mundaca-Moraga et al., 2021). Additionally, since the intensity of the multiple stressors (e.g., climate-change effects, habitat loss and degradation, eutrophication, introduction of alien species, fishing practices) is increasing throughout most of the Mediterranean basin, trend analysis is an essential process in assessing the state of the ocean of a region. This will contribute to effectively informing current and future marine policies and management actions as well as underpinning longer-term scientific objectives.
- k.
Extend the Mediterranean HFR time series. This would enable the widespread implementation of novel data science methodologies. It is expected that the HFR measurements will be expanded in the next decade in both space (i.e., new HFR systems) and time (i.e., longer time series). This will increase the availability of multiyear surface current datasets, thus contributing to boosting the application of HFR-derived STPs that use self-learning algorithms to other Mediterranean locations. For such sites, the stability of SOM solutions in time may be tested as well, or the self-learning and training of SOM solutions might change in time to properly reflect long-term changes in oceanographic conditions in a coastal area. The further development of short-term predictive systems based upon HFR surface current fields and their adaptation to the Mediterranean HFR network by incorporating non-tidal components of currents will enhance STP integration into operational maritime safety applications, which has demonstrated their capacity to reduce the searching area (Roarty et al., 2010).
- l.
Regionally contribute to long-term major effort. Building a sustained and fit-for-purpose European Ocean Observing System capable of supporting the UN Decade of Ocean Science for Sustainable Development and the European Green Deal should be twofold: (i) on the one hand, the Mediterranean HFR network outcomes should be scientifically grounded to further ensure the extension of science-based added-value products to societally relevant downstream services (Tintoré et al., 2019). (ii) On the other hand, the Mediterranean HFR community's long-standing cooperation must be further strengthened towards a co-designed and sustained regional network, contributing to and, simultaneously, being benefited by the European HFR Task Team (Corgnati et al., 2021; Rubio et al., 2021) endorsement, roadmap and main achievements (as recommended by Lorente et al., 2022).
The socioeconomically vital and environmentally stressed coastal areas of the Mediterranean Sea are some of the most exposed regions in the world due to the impact of climate change, also being highly vulnerable target regions for maritime safety, oil and marine litter pollution, fish stock overexploitation, and met-ocean hazards. The high spatiotemporal variability of the coastal dynamics requires the monitoring of these (sub-)mesoscale processes at the right scale. HFRs are currently the only technology for continuously monitoring surface currents (increasingly waves and winds) at unprecedentedly high spatiotemporal resolution over coastal areas and with relatively low cost and effort when compared with other traditional ocean observing platforms. Their integration in ocean observing has boosted progress in research on small-scale features and their interaction with larger scales, also underpinning the further development of applications. In this work, we present a review of the existing mature and emerging scientific and societal applications using HFR data, developed to address the major challenges identified in the Mediterranean coastal waters, organized around three main topics: (i) maritime safety, (ii) extreme hazards and (iii) environmental transport processes. In addition to previous studies carried out at global and European scale on this topic, this work also provides a list of strengths, weaknesses, opportunities and threats of the existing HFR applications in the Mediterranean Sea. Finally, we discuss the prospects for the future of HFR applications, and we provide a set of recommendations aiming to maximize the contribution to extending science-based HFR products to societally relevant downstream services (Tintoré et al., 2019) to support blue growth in the Mediterranean coastal areas, helping to meet the UN's Decade of Ocean Science for Sustainable Development and EU's Green Deal goals.
Considering the steadily growing integration of HFRs in the COOSs of the Mediterranean Sea and once their capabilities as an active and expanding field of investigation has been demonstrated by a wide range of practical applications, we can conclude that this consolidated land-based remote sensing technology plays a key role in the development of fit-for-purpose services for marine and maritime end users. However, major efforts should still be made to unlock HFR interoperable data access and potential as well as to further develop HFR scientific and societal applications at the regional level, thus delivering greater uptake, use and value. Fortunately, the opportunities provided in the framework of the UN Decade of Ocean Science for Sustainable Development and the European Green Deal can help to ensure the full exploitation of this HFR potential that will contribute to further deepening our understanding of coastal ocean dynamics and supporting sustainable blue growth in the Mediterranean Sea. In this sense, collaboration at regional level is crucial to address region-specific processes towards a fit-for-purpose and coordinated design of monitoring actions, to identify the environmental threats and their impacts on the environment and on blue sectors, to easily identify the existing stakeholders, also fostering interaction with them, and to engage potential users. This will help improve long-term sustainability together with training activities for the next generations and the search for funding. Certainly, this regional approach to strengthen collaborations should always be aligned with global and European strategies to ensure integration, benefiting from the European HFR roadmap and the availability of near-real-time and long-term HFR interoperable data that will boost research and underpin the further development of HFR scientific and societal applications in the Mediterranean coastal areas.
This paper constitutes the second part of a double contribution. Both parts support each other in an integrative way and should be interpreted as a single entity. The first part from Lorente et al. (2022) provides a comprehensive overview of the current status, achievements, challenges, coordinated efforts and roadmap to transform individual HFR systems into a fully integrated HFR network in the Mediterranean. Additionally, this work shows how these joint efforts have benefited and boosted HFR data integration into services and the development of a broad range of multidisciplinary science-based and fit-for-purpose applications, contributing to leveraging the HFR data to their fullest potential.
The European HFR node shares the code to process native HFR data for quality control and to convert them to the standard format for distribution for the workflows of both near-real-time and reprocessed data, as defined in Corgnati et al. (2020, 2019), respectively.
SOCIB HFR-Ibiza data, used in Sect. 2.1.1 (Fig. 2), Sect. 2.1.2 (Fig. 5) and Sect. 2.3.1 (Figs. 10 and 11), are available at http://apps.socib.es/data-catalog/#/data-products/hf-radar-ibiza (Tintoré et al., 2020). SOCIB drifter data, used in Sect. 2.1.1, are available at http://apps.socib.es/data-catalog/#/data-products/socib-exp-radar-sep2014 (Tintoré et al., 2014) for 2014 (they have also been used in Sect. 2.1.2, Fig. 5), http://apps.socib.es/data-catalog/#/data-products/socib-int-radarapm-jul2016 (Reyes et al., 2020c) for 2016 and http://apps.socib.es/data-catalog/#/data-products/socib-enl-lagrangian-nov2018 (Reyes et al., 2020b) for 2018. MIO's HFR-Toulon data is available http://hfradar.univ-tln.fr/HFRADAR/squel.php?content=accueil (last access: 5 May 2022, Dumas et al., 2022a) and real-time total currents (hourly data) in standard format are available for 2020 and 2021 in https://erddap.osupytheas.fr/erddap/files/cmems_nc_cf0e_c84a_8ead/ (last access: 5 May 2022, Dumas et al., 2022b)
The supplement related to this article is available online at: https://doi.org/10.5194/os-18-797-2022-supplement.
ER and PL conceived the idea of this paper and fostered the collaboration as MONGOOS HFR Task Team co-chairs, being in charge of overall direction and planning. All authors contributed to the writing of the different sections of the paper, as follows. ER took the lead in writing the Abstract and Sects. 1, 3, 4 and 5, also contributing to Sect. 2.1. Other authors contributed to Sect. 1 (CAG, DD, MB, SS) and Sect. 4 (YT, MB, RG). MB also contributed to the summary of HFR capabilities in Sects. 3 and 5. CRS, VC, ML, HM, AD, AG, AM, AR and ER (designed Fig. 1) collected the information from the maritime safety and rescue agencies for their respective countries. CRS and ML (designed Fig. 3) contributed to the introduction of Sect. 2.1, and, together with VC, AR (designed Fig. 2), JT and ER, they shaped Sect. 2.1.1. PL (designed Fig. 4), BM, JHL (designed Fig. 5), EA and ER wrote Sect. 2.1.2. IHC, AO, HM (designed Fig. 6), IV and VD took the lead in writing Sect. 2.1.3. MB (designed Fig. 7), AG, LC and CM contributed to the data analysis and text drafting in Sect. 2.2.1, in particular for the case study in the Ligurian Sea. In this section, PL (designed Fig. 8) and ER addressed two case studies in the Ebro Delta and one in the Strait of Gibraltar. CAG took the lead in writing Sect. 2.2.2, to which BM, ML and MJF also contributed. AM, ACE, IHC (designed Figs. 10 and 11) and AO contributed to the writing of Sect. 2.3.1. MBen – Consorzio LaMMA (designed Figs. 12 and 13), CB, ST, BD, MU, MJF, PF, EZ, HM and IV wrote Sect. 2.3.2. MM, DC (designed Fig. 14), RS (designed Fig. 15), FC (designed Fig. 16), CAG, MB, AG, IH and AO contributed to Sect. 2.3.3. Particularly, RS and MM focused on the Gulf of Manfredonia, DC on the Gulf of Naples, and FC on the Malta–Sicily case studies. AM, IV, CAG, JT and the MONGOOS co-chairs, VC and AO, provided critical feedback and helped shape the final version of the paper during the internal review process. All authors have read and agreed to the submission of the paper for publication.
Authors Maria Fattorini, Roberto Gómez and Pablo Lorente are currently employed at Qualitas Instruments Lda, at Helzel Messtechnik GmbH and at NOLOGIN Consulting SL, respectively. However, the authors have not advertised commercial products, and the research has not been sponsored by any one of the companies. At least one of the authors is a guest member of the editorial board of the special issue of Ocean Science. The peer-review process was guided by an independent editor, and the authors also have no other competing interests to declare.
Publisher's note: Copernicus Publications remains neutral with regard to jurisdictional claims in published maps and institutional affiliations.
This article is part of the special issue “Advances in interdisciplinary studies at multiple scales in the Mediterranean Sea”. It is a result of the 8th MONGOOS Meeting & Workshop, Trieste, Italy, 3–5 December 2019.
This work has been possible thanks to the MONGOOS (Mediterranean Operational Network for the Global Ocean Observing System) collaborative network, aimed toward long-term synergies between multidisciplinary working groups in the Mediterranean Sea in order to launch strategic initiatives and pursue funding for innovative research projects. Pierpaolo Falco and Enrico Zambianchi acknowledge partial funding from the Italian Ministry for University and Research through the 2017 PRIN project “Exploring the fate of Mediterranean microplastics: from distribution pathways to biological effects” (EMME, grant agreement no. 2017WERYZP). We are furthermore grateful for the valuable information about maritime rescue activity for 2019 provided by the Spanish Maritime Search and Rescue Agency, the Italian coast guard, the French CROSSMED, the Ministry of Maritime Affairs, Transport and Infrastructure from Croatia, and the search and rescue coordination centers from the SRRs (search and rescue regions) of Slovenia and Malta. We also acknowledge the Spanish Meteorological Agency AEMET for providing HARMONIE atmospheric fields and the European High-Frequency Radar Node (EU HFR NODE) for providing high-frequency radar surface current data from European and US radial sites at https://thredds.hfrnode.eu.html (last access: 5 May 2022). SOCIB's WMOP simulations are available upon request to info@socib.es and can be viewed at https://www.socib.es/?seccion=modelling&facility=forecast (last access: 16 May 2022). HFR-LaMMA data, used in Sect. 2.3.2, can be viewed at http://www.lamma.rete.toscana.it/meteo/osservazioni-e-dati/radar-hf, and HFR-NAdr data, used in Sect. 2.1.1. (Fig. 3), can be viewed at http://www.nib.si/mbp/en/oceanographic-data-and-measurements/. Data from the International Maritime Organization, mentioned in Sect. 1, are available from the Flow Monitoring Displacement Tracking Matrix website: https://migration.iom.int/europe?type=arrivals (last access: 16 May 2022). Eurostat statistics for maritime transport of goods, mentioned in the Introduction, are available at https://ec.europa.eu/eurostat/ (last access: 16 May 2022). The “Sub-regional Mediterranean Sea Indicators” tool, mentioned in the Sect. 1, is available at the following website: https://apps.socib.es/subregmed-indicators/ (last access: 16 May 2022). The SAR incidences in France, included in Sect. 2.1.1, were obtained from the website of the French Ministry of the Sea: https://www.mer.gouv.fr/surveillance-et-sauvetage-en-mer (last access: 16 May 2022).
The authors would like to thank Stephan Grilli from the University of Rhode Island (USA) for the design of Fig. 9 (from Sect. 2.2.2) and Leonardo Bagaglini for processing the data shown in Fig. 12 (from Sect. 2.3.2). Finally, the authors would like to express their gratitude to the internal reviewers, Anne Molcard, Ivica Vilibic, Charles-Antoine Guèrin and Joaquín Tintoré, as well as to the MONGOOS co-chairs, Vanessa Cardin and Alejandro Orfila, for their careful and meticulous reading of the paper. Their detailed and comprehensive reviews have been very helpful to improve the structure and reading as well as to finalize the paper.
Publication fees are provided by EuroSea (EU Horizon 2020 research and innovation program, grant agreement ID no. 862626) and JERICO-S3 (EU Horizon 2020 research and innovation program, grant agreement ID no. 871153). Research was supported by the following projects: CMEMS-INSTAC phase II, which provides the context of the activities for HFR data harmonization, standardization and distribution; the IBISAR CMEMS User-Uptake project (67-UU-DO-CMEMS-DEM4_LOT7); MEDCLIC project (LCF/PR/PR14/11090002, supported by La Caixa Foundation) that contributed to the development of the WMOP model; the CARTHE III project (Prime Award no. SA 18-14, subcontract agreement SPC-000649) and CALYPSO Departmental Research Initiative (grant no. N00014-18-1-2782), which supported the development of the methodology for extreme event monitoring in the Ligurian Sea (Sect. 2.2.1); the IMPACT project (EU funded, PC Interreg VA IFM 2014–2020, Prot. ISMAR no. 0002269) that funded the HFR network in the Ligurian Sea; the JERICO-NEXT project (EU Horizon 2020, grant agreement no. 654410) under which the assimilation of HFR data in WMOP (Sect. 2.1.2) and the biological connectivity application in the Gulf of Manfredonia (Sect. 2.3.3) have been developed; the COCONET project (EU FP7, grant agreement no. 287844) and the Italian national projects SSDPESCA and RITMARE; and the SICOMAR-PLUS EU Interreg Marittimo project, which funded the recent upgrades to the HFR installations of the MIO in Toulon.
This paper was edited by Anna Rubio and reviewed by two anonymous referees.
Abascal, A. J., Castanedo, S., Medina, R., Losada, I. J., and Álvarez-Fanjul, E.: Application of HF radar currents to oil spill modelling, Mar. Pollut. Bull., 58, 238–248, https://doi.org/10.1016/j.marpolbul.2008.09.020, 2009.
Abascal, A. J., Castanedo, S., Fernández, V., and Medina, R.: Backtracking drifting objects using surface currents from high-frequency (HF) radar technology, Ocean Dynam., 62, 1073–1089, https://doi.org/10.1007/s10236-012-0546-4, 2012.
Abascal, A. J., Sánchez, J., Chiri, H., Ferrer, M. I., Cárdenas, M., Gallego, A., Castanedo, S., Medina, R., Alonso-Martirena, A., Berx, B., Turrell, W. R., and Hughes, S. L.: Operational oil spill trajectory modelling using HF radar currents: A northwest European continental shelf case study, Mar. Pollut. Bull., 119, 336–350, https://doi.org/10.1016/j.marpolbul.2017.04.010, 2017.
Aguiar, E., Mourre, B., Juza, M., Reyes, E., Hernández-Lasheras, J., Cutolo, E., Mason, E., and Tintoré, J.: Multi-platform model assessment in the Western Mediterranean Sea: impact of downscaling on the surface circulation and mesoscale activity, Ocean Dynam., 70, 273–288, https://doi.org/10.1007/s10236-019-01317-8, 2020.
Allou, A., Forget, P., and Devenon, J.-L.: Submesoscale vortex structures at the entrance of the Gulf of Lions in the Northwestern Mediterranean Sea, Cont. Shelf Res., 30, 724–732, https://doi.org/10.1016/j.csr.2010.01.006, 2010.
Álvarez-Fanjul, E., Gómez, B. P., and Arevalo, I. R. S.: Nivmar: a storm surge forecasting system for Spanish waters, Sci. Mar., 65, 145–154, 2001.
Alvera-Azcárate, A., Barth, A., Rixen, M., and Beckers, J.-M.: Reconstruction of incomplete oceanographic data sets using Empirical Orthogonal Functions, Application to the Adriatic Sea, Ocean Model., 9, 325–346, 2005
Amores, A., Marcos, M., Carrió, D. S., and Gómez-Pujol, L.: Coastal impacts of Storm Gloria (January 2020) over the north-western Mediterranean, Nat. Hazards Earth Syst. Sci., 20, 1955–1968, https://doi.org/10.5194/nhess-20-1955-2020, 2020.
Archer, M. R., Roughan, M., Keating, S. R., and Schaeffer, A.: On the Variability of the East Australian Current: Jet Structure, Meandering, and Influence on Shelf Circulation, J. Geophys. Res.-Oceans, 122, 8464–8481, https://doi.org/10.1002/2017JC013097, 2017.
Arunraj, K. S., Jena, B. K., Suseentharan, V., and Rajkumar, J.: Variability in Eddy Distribution Associated With East India Coastal Current From High-Frequency Radar Observations Along Southeast Coast of India, J. Geophys. Res.-Oceans, 123, 9101–9118, https://doi.org/10.1029/2018JC014041, 2018.
Astraldi, M. and Gasparini, G. P.: The seasonal characteristics of the circulation in the north Mediterranean basin and their relationship with the atmospheric-climatic conditions, J. Geophys. Res.-Oceans, 97, 9531–9540, https://doi.org/10.1029/92JC00114, 1992.
Bagaglini, L., Falco, P., and Zambianchi, E.: Eddy Detection in HF Radar-Derived Surface Currents in the Gulf of Naples, Remote Sens., 12, 97, https://doi.org/10.3390/rs12010097, 2020.
Barrick, D. E.: A coastal radar system for tsunami warning, Remote Sens. Environ., 8, 353–358, https://doi.org/10.1016/0034-4257(79)90034-8, 1979.
Barrick, D. and Lipa, B.: Correcting for distorted antenna patterns in CODAR ocean surface measurements, IEEE J. Ocean. Eng., 11, 304–309, https://doi.org/10.1109/JOE.1986.1145158, 1986.
Barrick, D., Fernandez, V., Ferrer, M. I., Whelan, C., and Breivik, Ø.: A short-term predictive system for surface currents from a rapidly deployed coastal HF radar network, Ocean Dynam., 62, 725–740, https://doi.org/10.1007/s10236-012-0521-0, 2012.
Barth, A., Alvera-Azcárate, A., and Weisberg, R. H.: Assimilation of high-frequency radar currents in a nested model of the West Florida Shelf, J. Geophys. Res.-Oceans, 113, 1–15, https://doi.org/10.1029/2007JC004585, 2008.
Barth, A., Troupin, C., Reyes, E., Alvera-Azcárate, A., Beckers, J.-M., and Tintoré, J.: Variational interpolation of high-frequency radar surface currents using DIVAnd, Ocean Dynam., 71, 293–308, https://doi.org/10.1007/s10236-020-01432-x, 2021.
Basañez, A., Lorente, P., Montero, P., Álvarez-Fanjul, E., and Pérez-Muñuzuri, V.: Quality assessment and practical interpretation of the wave parameters estimated by HF radars in NW Spain, Remote Sens., 12, 1–24, https://doi.org/10.3390/rs12040598, 2020.
Basañez, A. and Pérez-Muñuzuri, V.: HF Radars for Wave Energy Resource Assessment Offshore NW Spain, Remote Sens., 13, https://doi.org/10.3390/rs13112070, 2021.
Beckers, J. M. and Rixen, M.: EOF Calculations and Data Filling from Incomplete Oceanographic Datasets, J. Atmos. Ocean. Technol., 20, 1839–1856, https://doi.org/10.1175/1520-0426(2003)020<1839:ECADFF>2.0.CO;2, 2003.
Behrenfeld, M. J., O'Malley, R. T., Siegel, D. A., McClain, C. R., Sarmiento, J. L., Feldman, G. C., Milligan, A. J., Falkowski, P. G., Letelier, R. M., and Boss, E. S.: Climate-driven trends in contemporary ocean productivity, Nature, 444, 752–755, https://doi.org/10.1038/nature05317, 2006.
Benaïchouche, S., Legoff, C., Guichoux, Y., Rousseau, F. and Fablet, R.: Unsupervised Reconstruction of Sea Surface Currents from AIS Maritime Traffic Data Using Trainable Variational Models, Remote Sens., 13, https://doi.org/10.3390/rs13163162, 2021.
Berta, M., Bellomo, L., Magaldi, M. G., Griffa, A., Molcard, A., Marmain, J., Borghini, M., and Taillandier, V.: Estimating Lagrangian transport blending drifters with HF radar data and models: Results from the TOSCA experiment in the Ligurian Current (North Western Mediterranean Sea), Prog. Oceanogr., 128, 15–29, https://doi.org/10.1016/j.pocean.2014.08.004, 2014a.
Berta, M., Ursella, L., Nencioli, F., Doglioli, A. M., Petrenko, A. A., and Cosoli, S.: Surface transport in the Northeastern Adriatic Sea from FSLE analysis of HF radar measurements, Cont. Shelf Res., 77, 14–23, https://doi.org/10.1016/j.csr.2014.01.016, 2014b.
Berta, M., Bellomo, L., Griffa, A., Magaldi, M. G., Molcard, A., Mantovani, C., Gasparini, G. P., Marmain, J., Vetrano, A., Béguery, L., Borghini, M., Barbin, Y., Gaggelli, J., and Quentin, C.: Wind-induced variability in the Northern Current (northwestern Mediterranean Sea) as depicted by a multi-platform observing system, Ocean Sci., 14, 689–710, https://doi.org/10.5194/os-14-689-2018, 2018.
Berta, M., Corgnati, L., Magaldi, M. G., Griffa, A., Mantovani, C., Rubio, A., Reyes, E., and Mader, J.: Small scale ocean weather during an extreme wind event in the Ligurian Sea, in: Copernicus Marine Service Ocean State Report, Issue 4, J. Oper. Oceanogr., 13, 149–155, https://doi.org/10.1080/1755876X.2020.1785097, 2020.
Bettencourt, J., López, C., Hernández-García, E., Montes, I., Sudre, J., Dewitte, B., Paulmier, A., and Garçon, V.: Boundaries of the Peruvianoxygen minimum zone shaped by coherent mesoscale dynamics, Nat. Geosci., 8, 937–940, https://doi.org/10.1038/ngeo2570, 2015.
Bjorkstedt, E. and Roughgarden, J.: Larval transport and coastal upwelling: An application of HF radar in ecological research, Oceanography, 10, 64–67, https://doi.org/10.5670/oceanog.1997.25, 1997.
Bolado-Penagos, M., Sala, I., Gomiz-Pascual, J. J., Romero-Cózar, J., González-Fernández, D., Reyes-Pérez, J., Vázquez, A., and Bruno, M.: Revising the effects of local and remote atmospheric forcing on the Atlantic Jet and Western Alboran Gyre dynamics, J. Geophys. Res.-Oceans, 126, e2020JC016173, https://doi.org/10.1029/2020JC016173, 2021.
Bourg, N. and Molcard, A.: Northern boundary current variability and mesoscale dynamics: a long-term HF RADAR monitoring in the North-Western Mediterranean Sea, Ocean Dynam., 71, 851–870, https://doi.org/10.1007/s10236-021-01466-9, 2021.
Breivik, Ø. and Satra, Ø.: Real time assimilation of HF radar currents into a coastal ocean model, J. Mar. Syst., 28, 161–182, https://doi.org/10.1016/S0924-7963(01)00002-1, 2001.
Breivik, Ø., Allen, A. A., Maisondieu, C., and Olagnon, M.: Advances in search and rescue at sea Topical Collection on Advances in Search and Rescue at Sea, Ocean Dynam., 63, 83–88, https://doi.org/10.1007/s10236-012-0581-1, 2013.
Brzezinski, M. A. and Washburn, L.: Phytoplankton primary productivity in the Santa Barbara Channel: Effects of wind-driven upwelling and mesoscale eddies, J. Geophys. Res.-Oceans, 116, C12013, https://doi.org/10.1029/2011JC007397, 2011.
Bué, I., Semedo, Á., and Catalão, J.: Evaluation of HF Radar Wave Measurements in Iberian Peninsula by Comparison with Satellite Altimetry and in Situ Wave Buoy Observations, Remote Sens., 12, 3623, https://doi.org/10.3390/rs12213623, 2020.
Caballero, A., Mulet, S., Ayoub, N., Manso-Narvarte, I., Davila, X., Boone, C., Toublanc, F., and Rubio, A.: Integration of HF Radar Observations for an Enhanced Coastal Mean Dynamic Topography, Front. Mar. Sci., 7, 588713, https://doi.org/10.3389/fmars.2020.588713, 2020.
Caldeira, R. M. A., Couvelard, X., Casella, E., and Vetrano, A.: Assymmetric eddy populations in adjacent basins – a high resolution numerical study of the Tyrrhenian and Ligurian Seas, Ocean Sci. Discuss., 9, 3521–3566, https://doi.org/10.5194/osd-9-3521-2012, 2012.
Candela, J., Mazzola, S., Sammari, C., Limeburner, R., Lozano, C. J., Patti, B., and Bonanno, A.: The “Mad Sea” Phenomenon in the Strait of Sicily, J. Phys. Oceanogr., 29, 2210–2231, https://doi.org/10.1175/1520-0485(1999)029<2210:TMSPIT>2.0.CO;2, 1999.
Capet, A., Fernández, V., She, J., Dabrowski, T., Umgiesser, G., Staneva, J., Mészáros, L., Campuzano, F., Ursella, L., Nolan, G., and El Serafy, G.: Operational Modeling Capacity in European Seas – An EuroGOOS Perspective and Recommendations for Improvement, Front. Mar. Sci., 7, 129, https://doi.org/10.3389/fmars.2020.00129, 2020.
Capodici, F., Ciraolo, G., Cosoli, S., Maltese, A., Mangano, M. C., and Sarà, G.: Downscaling hydrodynamics features to depict causes of major productivity of Sicilian-Maltese area and implications for resource management, Sci. Total Environ., 628, 815–825, https://doi.org/10.1016/j.scitotenv.2018.02.106, 2018.
Charney, J. G.: Geostrophic Turbulence, J. Atmos. Sci., 28, 1087–1095, https://doi.org/10.1175/1520-0469(1971)028<1087:GT>2.0.CO;2, 1971.
Chelton, D. B., Schlax, M. G., and Samelson, R. M.: Global observations of nonlinear mesoscale eddies, Prog. Oceanogr., 91, 167–216, https://doi.org/10.1016/j.pocean.2011.01.002, 2011.
Cianelli, D., Uttieri, M., Guida, R., Menna, M., Buonocore, B., Falco, P., Zambardino, G., and Zambianchi, E.: Land-based remote sensing of coastal basins: Use of an HF radar to investigate surface dynamics and transport processes in the Gulf of Naples, in: Remote Sensing: Techniques, Appl. Tech., 1–30, 2013.
Cianelli, D., D'Alelio, D., Uttieri, M., Sarno, D., Zingone, A., Zambianchi, E., and D'Alcalà, M. R.: Disentangling physical and biological drivers of phytoplankton dynamics in a coastal system, Sci. Rep., 7, 1–15, https://doi.org/10.1038/s41598-017-15880-x, 2017.
Ciuffardi, T., Napolitano, E., Iacono, R., Reseghetti, F., Raiteri, G., and Bordone, A.: Analysis of surface circulation structures along a frequently repeated XBT transect crossing the Ligurian and Tyrrhenian Seas, Ocean Dynam., 66, 767–783, https://doi.org/10.1007/s10236-016-0954-y, 2016.
Cohuet, J. B., Romero, R., Homar, V., Ducrocq, V., and Ramis, C.: Initiation of a severe thunderstorm over the Mediterranean Sea, Atmos. Res., 100, 603–620, https://doi.org/10.1016/j.atmosres.2010.11.002, 2011.
Coll, M., Piroddi, C., Steenbeek, J., Kaschner, K., Ben Rais Lasram, F., Aguzzi, J., Ballesteros, E., Bianchi, C. N., Corbera, J., Dailianis, T., Danovaro, R., Estrada, M., Froglia, C., Galil, B. S., Gasol, J. M., Gertwagen, R., Gil, J., Guilhaumon, F., Kesner-Reyes, K., Kitsos, M.-S., Koukouras, A., Lampadariou, N., Laxamana, E., López-Fé de la Cuadra, C. M., Lotze, H. K., Martin, D., Mouillot, D., Oro, D., Raicevich, S., Rius-Barile, J., Saiz-Salinas, J. I., San Vicente, C., Somot, S., Templado, J., Turon, X., Vafidis, D., Villanueva, R., and Voultsiadou, E.: The Biodiversity of the Mediterranean Sea: Estimates, Patterns, and Threats, PLoS One, 5, e11842, https://doi.org/10.1371/journal.pone.0011842, 2010.
Conti, D., Orfila, A., Mason, E., Sayol, J. M., Simarro, G., and Balle, S.: An eddy tracking algorithm based on dynamical systems theory, Ocean Dynam., 66, 1415–1427, https://doi.org/10.1007/s10236-016-0990-7, 2016.
Corgnati, L.: LorenzoCorgnati/HFR_Node_Historical_Data_Processing: EU_HFR_NODE_Historical_Data_Processing (Version v2.1.1.6). Zenodo [code], https://doi.org/10.5281/zenodo.3569519, last access: 10 December 2019.
Corgnati, L.: LorenzoCorgnati/HFR_Node_tools: EU_HFR_NODE_Tools (Version v2.1.2), Zenodo [code], https://doi.org/10.5281/zenodo.3855461, last access: 26 May 2020.
Corgnati, L., Mantovani, C., Novellino, A., Rubio, A., and Mader, J.: Recommendation Report 2 on improved common procedures for HFR QC analysis. JERICO-NEXT WP5-Data Management, Deliverable 5.14, Version 1.0, Brest, France, IFREMER, 82 pp., (JERICO-NEXT-WP5-D5.14-V1.), https://doi.org/10.25607/OBP-944, 2018.
Corgnati, L., Mantovani, C., Griffa, A., Berta, M., Penna, P., Celentano, P., Bellomo, L., Carlson, D. F., and D'Adamo, R.: Implementation and Validation of the ISMAR High-Frequency Coastal Radar Network in the Gulf of Manfredonia (Mediterranean Sea), IEEE J. Ocean. Eng., 44, 424–445, https://doi.org/10.1109/JOE.2018.2822518, 2019a.
Corgnati, L., Mantovani, C., Novellino, A., Jousset, S., Cramer, R. N., and Thijsse, P.: SeaDataNet data management protocols for HF Radar data, WP9 – Deliverable D9.12. Version 1.6. SeaDataNet, 83 pp., https://doi.org/10.25607/OBP-1011, 2019b.
Corgnati, L., Mantovani, C., Rubio, A., Reyes, E., Rotllan, P., Novellino, A., Gorringe, P., Solabarrieta, L., Griffa, A., and Mader, J.: The Eurogoos High Frequency radar task team: a success story of collaboration to be kept alive and made growing, in 9th EuroGOOS International conference, 467–474, Brest, France, https://hal.archives-ouvertes.fr/hal-03328829 (last access: 16 May 2022), 2021.
Coulliette, C., Lekien, F., Paduan, J. D., Haller, G., and Marsden, J. E.: Optimal Pollution Mitigation in Monterey Bay Based on Coastal Radar Data and Nonlinear Dynamics, Environ. Sci. Technol., 41, 6562–6572, https://doi.org/10.1021/es0630691, 2007.
Cózar, A., Sanz-Martín, M., Martí, E., González-Gordillo, J. I., Ubeda, B., Gálvez, J. Á., Irigoien, X., and Duarte, C. M.: Plastic Accumulation in the Mediterranean Sea, PLoS One, 10, e0121762, https://doi.org/10.1371/journal.pone.0121762, 2015.
Davidson, F., Alvera-Azcárate, A., Barth, A., Brassington, G. B., Chassignet, E. P., Clementi, E., De Mey-Frémaux, P., Divakaran, P., Harris, C., Hernandez, F., Hogan, P., Hole, L. R., Holt, J., Liu, G., Lu, Y., Lorente, P., Maksymczuk, J., Martin, M., Mehra, A., Melsom, A., Mo, H., Moore, A., Oddo, P., Pascual, A., Pequignet, A.-C., Kourafalou, V., Ryan, A., Siddorn, J., Smith, G., Spindler, D., Spindler, T., Stanev, E. V, Staneva, J., Storto, A., Tanajura, C., Vinayachandran, P. N., Wan, L., Wang, H., Zhang, Y., Zhu, X., and Zu, Z.: Synergies in Operational Oceanography: The Intrinsic Need for Sustained Ocean Observations, Front. Mar. Sci., 6, 450, https://doi.org/10.3389/fmars.2019.00450, 2019.
Davila, X., Rubio, A., Artigas, L. F., Puillat, I., Manso-Narvarte, I., Lazure, P., and Caballero, A.: Coastal submesoscale processes and their effect on phytoplankton distribution in the southeastern Bay of Biscay, Ocean Sci., 17, 849–870, https://doi.org/10.5194/os-17-849-2021, 2021.
De Alfonso, M., Lin-Ye, J., García-Valdecasas, J. M., Pérez-Rubio, S., Luna, M. Y., Santos-Muñoz, D., Ruiz, M. I., Pérez-Gómez, B., and Álvarez-Fanjul, E.: Storm Gloria: Sea State Evolution Based on in situ Measurements and Modeled Data and Its Impact on Extreme Values, Front. Mar. Sci., 8, 270, https://doi.org/10.3389/fmars.2021.646873, 2021.
De Dominicis, M., Pinardi, N., Zodiatis, G., and Lardner, R.: MEDSLIK-II, a Lagrangian marine surface oil spill model for short-term forecasting – Part 1: Theory, Geosci. Model Dev., 6, 1851–1869, https://doi.org/10.5194/gmd-6-1851-2013, 2013a.
De Dominicis, M., Pinardi, N., Zodiatis, G., and Archetti, R.: MEDSLIK-II, a Lagrangian marine surface oil spill model for short-term forecasting – Part 2: Numerical simulations and validations, Geosci. Model Dev., 6, 1871–1888, https://doi.org/10.5194/gmd-6-1871-2013, 2013b.
De Mey-Frémaux, P., Ayoub, N., Barth, A., Brewin, R., Charria, G., Campuzano, F., Ciavatta, S., Cirano, M., Edwards, C. A., Federico, I., Gao, S., Garcia Hermosa, I., Garcia Sotillo, M., Hewitt, H., Hole, L. R., Holt, J., King, R., Kourafalou, V., Lu, Y., Mourre, B., Pascual, A., Staneva, J., Stanev, E. V, Wang, H., and Zhu, X.: Model-Observations Synergy in the Coastal Ocean, Front. Mar. Sci., 6, 436, https://doi.org/10.3389/fmars.2019.00436, 2019.
Declerck, A., Delpey, M., Rubio, A., Ferrer, L., Basurko, O. C., Mader, J., and Louzao, M.: Transport of floating marine litter in the coastal area of the south-eastern Bay of Biscay: A Lagrangian approach using modelling and observations, J. Oper. Oceanogr., 12, 111–125, https://doi.org/10.1080/1755876X.2019.1611708, 2019.
Denamiel, C., Šepić, J., Ivanković, D., and Vilibić, I.: The Adriatic Sea and Coast modelling suite: Evaluation of the meteotsunami forecast component, Ocean Model., 135, 71–93, https://doi.org/10.1016/j.ocemod.2019.02.003, 2019.
Di Muccio, S., Rak, G., Giordano, P., Mannozzi, M., Sammarini, V., Alcaro, L., de la Fuente Origlia, A., Amato, E., and Renzi, P.: Quaderni delle Emergenze Ambientali in Mare. 05-Modalità di campionamento degli idrocarburi in mare e lungo la costa, La valutazione della contaminazione del litorale a seguito di oil spill, ISBN 978-88-448-0579-1, 2020.
Domps, B., Marmain, J. and Guèrin, C.-A.: A Reanalysis of the October 2016 “Meteotsunami” in British Columbia With Help of High-Frequency Radars and Autoregressive Modeling, arXiv Atmos. Ocean. Phys., https://doi.org/10.48550/arXiv.2011.07237, 2020.
Dong, C., McWilliams, J. C., Liu, Y., and Chen, D.: Global heat and salt transports by eddy movement, Nat. Commun., 5, 3294, https://doi.org/10.1038/ncomms4294, 2014.
Drago, A.: Numerical modelling of coastal seiches in Malta, Phys. Chem. Earth, 33, 260–275, https://doi.org/10.1016/j.pce.2007.02.001, 2008.
Dumas, D. and Guérin, C.-A.: Self-calibration and antenna grouping for bistatic oceanographic High-Frequency Radars, Electr. Eng. Sys. Sci., https://doi.org/10.48550/arXiv.2005.10528, 2020.
Dumas, D., Guérin, C.-A., Quentin, C., and Molcard, A.: MIO's HFRToulon data, Mediterranean Institute of Oceanography, [data set], http://hfradar.univ-tln.fr/HFRADAR/squel.php?content=accueil, last access: 5 May 2022a.
Dumas, D., Quentin, C., and Guérin, C.-A.: Real time total currents foe 2020 and 2021, Mediterranean Institute of Oceanography, [data set], https://erddap.osupytheas.fr/erddap/files/cmems_nc_cf0e_c84a_8ead/, last access: 5 May 2022b.
Dzvonkovskaya, A.: Ocean surface current measurements using HF radar during the 2011 Japan tsunami hitting Chilean coast, 2012 IEEE Int. Geosci. Remote Sens. Symp., 7605–7608, 2012.
Dzvonkovskaya, A. L. and Rohling, H.: HF radar ship detection and tracking using WERA system, in 2007 IET International Conference on Radar Systems, 1–5, https://doi.org/10.1049/cp:20070478, 2007.
Dzvonkovskaya, A., Petersen, L., and Insua, T. L.: Real-time capability of meteotsunami detection by WERA ocean radar system, in 2017 IEEE18th International Radar Symposium (IRS), 1–10, https://doi.org/10.23919/IRS.2017.8008096, 2017.
Ebling, J. and Scheuermann, G.: Clifford convolution and pattern matching on vector fields, in: IEEE Visualization, 2003, VIS 2003, 193–200, https://doi.org/10.1109/VISUAL.2003.1250372, 2003.
European Commission: The EU Blue Economy Report 2020. Publications Office of the European Union, Luxembourg, https://op.europa.eu/s/u0bL (last access: 9 May 2022), 2020.
Falco, P., Buonocore, B., Cianelli, D., De Luca, L., Giordano, A., Iermano, I., Kalampokis, A., Saviano, S., Uttieri, M., Zambardino, G., and Zambianchi, E.: Dynamics and sea state in the Gulf of Naples: potential use of high-frequency radar data in an operational oceanographic context, J. Oper. Oceanogr., 9, 33–45, https://doi.org/10.1080/1755876X.2015.1115633, 2016.
Fang, F. and Morrow, R.: Evolution, movement and decay of warm-core Leeuwin Current eddies, Deep Sea Res. Pt. II, 50, 2245–2261, https://doi.org/10.1016/S0967-0645(03)00055-9, 2003.
Fossi, M. C., Romeo, T., Baini, M., Panti, C., Marsili, L., Campani, T., Canese, S., Galgani, F., Druon, J.-N., Airoldi, S., Taddei, S., Fattorini, M., Brandini, C., and Lapucci, C.: Plastic Debris Occurrence, Convergence Areas and Fin Whales Feeding Ground in the Mediterranean Marine Protected Area Pelagos Sanctuary: A Modeling Approach, Front. Mar. Sci., 4, 167, https://doi.org/10.3389/fmars.2017.00167, 2017.
Fox-Kemper, B., Adcroft, A., Böning, C. W., Chassignet, E. P., Curchitser, E., Danabasoglu, G., Eden, C., England, M. H., Gerdes, R., Greatbatch, R. J., Griffies, S. M., Hallberg, R. W., Hanert, E., Heimbach, P., Hewitt, H. T., Hill, C. N., Komuro, Y., Legg, S., Le Sommer, J., Masina, S., Marsland, S. J., Penny, S. G., Qiao, F., Ringler, T. D., Treguier, A. M., Tsujino, H., Uotila, P., and Yeager, S. G.: Challenges and Prospects in Ocean Circulation Models, Front. Mar. Sci., 6, 65, https://doi.org/10.3389/fmars.2019.00065, 2019.
Frolov, S., Paduan, J., Cook, M., and Bellingham, J.: Improved statistical prediction of surface currents based on historic HF-radar observations, Ocean Dynam., 62, 1111–1122, https://doi.org/10.1007/s10236-012-0553-5, 2012.
Fujii, S., Heron, M. L., Kim, K., Lai, J.-W., Lee, S.-H., Wu, X., Wu, X., Wyatt, L. R., and Yang, W.-C.: An overview of developments and applications of oceanographic radar networks in Asia and Oceania countries, Ocean Sci. J., 48, 69–97, https://doi.org/10.1007/s12601-013-0007-0, 2013.
Gabrié, C., Lagabrielle, E., Bissery, C., Crochelet, E., Meola, B., Webster, C., Claudet, J., Chassanite, A., Marinesque, S., Robert, P., Goutx, M., and Quod, C.: The Status of Marine Protected Areas in the Mediterranean Sea. MedPAN & RAC/SPA., MedPAN Collection, http://www.rac-spa.org/sites/default/files/ (last access: 13 May 2022), 2012.
Garcıa-Lafuente, J., Delgado, J., and Criado, F.: Inflow interruption by meteorological forcing in the Strait of Gibraltar, Geophys. Res. Lett., 29, 20–24, https://doi.org/10.1029/2002GL015446, 2002.
García-Sánchez, G., Mancho, A. M., Ramos, A. G., Coca, J., and Wiggins, S.: Structured pathways in the turbulence organizing recent oil spill events in the Eastern Mediterranean, Sci. Rep., 12, 3662, https://doi.org/10.1038/s41598-022-07350-w, 2022.
Garrett, C. J. R.: Variable sea level and strait flows in the Mediterranean: a theoretical study of the response to meteorological forcing, Ocean. Ac., 6, 79–88, 1983.
Gildor, H., Fredj, E., Steinbuck, J., and Monismith, S.: Evidence for Submesoscale Barriers to Horizontal Mixing in the Ocean from Current Measurements and Aerial Photographs, J. Phys. Oceanogr., 39, 1975–1983, https://doi.org/10.1175/2009JPO4116.1, 2009.
Gómez-Navarro, L., Fablet, R., Mason, E., Pascual, A., Mourre, B., Cosme, E., and Le Sommer, J.: SWOT Spatial Scales in the Western Mediterranean Sea Derived from Pseudo-Observations and an Ad Hoc Filtering, Remote Sens., 10, 599, https://doi.org/10.3390/rs10040599, 2018.
Gómez, M., Álvarez-Fanjul, E., Carretero, J. C., Pérez-Gómez, B., Rodríguez, I., Serrano, O., and Sotillo, M. G.: Oceanographic and atmospheric analysis of the 10–16 November 2001 Storm in the Western Mediterranean, in 4th EGS 85 Plinius Conference on Mediterranean Storms, p. 5, Universitat de les Illes Balears, Mallorca, Spain, http://meteorologia.uib.eu/ROMU/informal/proceedings_4th_plinius_02/PDFs/Gomez_et_al.pdf (last access: 19 May 2022), 2002.
Gommenginger, C., Martin, A. C. H., Jacob, B., and Staneva, J.: Multi-year assessment of ocean surface currents from Copernicus Sentinel-1 and HF radar in the German Bight, EGU General Assembly 2021, online, 19–30 April 2021, EGU21-15280, https://doi.org/10.5194/egusphere-egu21-15280, 2021.
Griffa, A., Horstmann, J., Mader, J., Rubio, A., Berta, M., Orfila, A., and Lars, A.: Report on final assessment of methodological improvements and testing, JERICO-NEXT WP3 Innovations in Technology and Methodology, Deliverable D3.4, Version 2, Brest, France, IFREMER, 56 pp. (JERICO-NEXT-WP3-D3.4-180719-V2), https://doi.org/10.25607/OBP-948, 2019.
Grilli, S. T., Grosdidier, S., and Guérin, C. A.: Tsunami Detection by High-Frequency Radar Beyond the Continental Shelf, in: Global Tsunami Science: Past and Future, edited by: Geist, E. L., Fritz, H. M., Rabinovich, A. B., and Tanioka, Y., Vol. I, Springer, 3895–3934, 2015.
Guérin, C.-A., Grilli, S., Moran, P., Grilli, A., and Insua, T.: Tsunami detection by high-frequency radar in British Columbia: performance assessment of the time-correlation algorithm for synthetic and real events, Ocean Dynam., 68, 423–438, https://doi.org/10.1007/s10236-018-1139-7, 2018.
Guihou, K., Marmain, J., Ourmieres, Y., Molcard, A., Zakardjian, B., and Forget, P.: A case study of the mesoscale dynamics in the North-Western Mediterranean Sea: a combined data-model approach, Ocean Dynam., 63, 793–808, https://doi.org/10.1007/s10236-013-0619-z, 2013.
Gurgel, K., Essen, H., and Schlick, T.: An Empirical Method to Derive Ocean Waves From Second-Order Bragg Scattering: Prospects and Limitations, IEEE J. Ocean. Eng., 31, 804–811, https://doi.org/10.1109/JOE.2006.886225, 2006.
Gurgel, K.-W., Dzvonkovskaya, A., Pohlmann, T., Schlick, T., and Gill, E.: Simulation and detection of tsunami signatures in ocean surface currents measured by HF radar, Ocean Dynam., 61, 1495–1507, https://doi.org/10.1007/s10236-011-0420-9, 2011.
Haller, G.: Lagrangian Coherent Structures, Annu. Rev. Fluid Mech., 47, 137–162, https://doi.org/10.1146/annurev-fluid-010313-141322, 2015.
Haza, A. C., Özgökmen, T. M., Griffa, A., Molcard, A., Poulain, P.-M., and Peggion, G.: Transport properties in small-scale coastal flows: relative dispersion from VHF radar measurements in the Gulf of La Spezia, Ocean Dynam., 60, 861–882, https://doi.org/10.1007/s10236-010-0301-7, 2010.
Headrick, J. M. and Thomason, J. F.: Applications of high-frequency radar, Radio Sci., 33, 1045–1054, https://doi.org/10.1029/98RS01013, 1998.
Heiberg, E., Ebbers, T., Wigstrom, L., and Karlsson, M.: Three-dimensional flow characterization using vector pattern matching, IEEE Trans. Vis. Comput. Graph., 9, 313–319, https://doi.org/10.1109/TVCG.2003.1207439, 2003.
Hernández-Carrasco, I., Rossi, V., Hernández-García, E., Garçon, V., and López, C.: The reduction of plankton biomass induced by mesoscale stirring: A modeling study in the Benguela upwelling, Deep Sea Res. Pt. I, 83, 65–80, https://doi.org/10.1016/j.dsr.2013.09.003, 2014.
Hernández-Carrasco, I., Orfila, A., Rossi, V., and Garçon, V.: Effect of small scale transport processes on phytoplankton distribution in coastal seas, Sci. Rep., 8, 1–13, https://doi.org/10.1038/s41598-018-26857-9, 2018a.
Hernández-Carrasco, I., Solabarrieta, L., Rubio, A., Esnaola, G., Reyes, E., and Orfila, A.: Impact of HF radar current gap-filling methodologies on the Lagrangian assessment of coastal dynamics, Ocean Sci., 14, 827–847, https://doi.org/10.5194/os-14-827-2018, 2018b.
Hernández-Carrasco, I., Alou-Font, E., Dumont, P.-A., Cabornero, A., Allen, J., and Orfila, A.: Lagrangian flow effects on phytoplankton abundance and composition along filament-like structures, Prog. Oceanogr., 189, 102469, https://doi.org/10.1016/j.pocean.2020.102469, 2020.
Hernández-Lasheras, J., Mourre, B., Orfila, A., Santana, A., Reyes, E., and Tintoré, J.: Evaluating High-Frequency radar data assimilation impact in coastal ocean operational modelling, Ocean Sci. Discuss., 2021, 1–29, https://doi.org/10.5194/os-2021-34, 2021.
Hernandez, F., Blockley, E., Brassington, G. B., Davidson, F., Divakaran, P., Drévillon, M., Ishizaki, S., Garcia-Sotillo, M., Hogan, P. J., Lagemaa, P., Levier, B., Martin, M., Mehra, A., Mooers, C., Ferry, N., Ryan, A., Regnier, C., Sellar, A., Smith, G. C., Sofianos, S., Spindler, T., Volpe, G., Wilkin, J., Zaron, E. D., and Zhang, A.: Recent progress in performance evaluations and near real-time assessment of operational ocean products, J. Oper. Oceanogr., 8, 221–238, https://doi.org/10.1080/1755876X.2015.1050282, 2015.
Heron, M. L.: Applying a unified directional wave spectrum to the remote sensing of wind wave directional spreading, Can. J. Remote Sens., 28, 346–353, https://doi.org/10.5589/m02-030, 2002.
Huang, W., Gill, E., Wu, S., Wen, B., Yang, Z., and Hou, J.: Measuring Surface Wind Direction by Monostatic HF Ground-Wave Radar at the Eastern China Sea, Ocean. Eng. IEEE J., 29, 1032–1037, https://doi.org/10.1109/JOE.2004.834175, 2004.
Iermano, I., Moore, A. M., and Zambianchi, E.: Impacts of a 4-dimensional variational data assimilation in a coastal ocean model of southern Tyrrhenian Sea, J. Mar. Syst., 154, 157–171, https://doi.org/10.1016/j.jmarsys.2015.09.006, 2016.
Isern-Fontanet, J., García-Ladona, E., and Font, J.: Identification of Marine Eddies from Altimetric Maps, J. Atmos. Ocean. Technol., 20, 772–778, https://doi.org/10.1175/1520-0426(2003)20< 772:IOMEFA>2.0.CO;2, 2003.
Janeković, I., Mihanović, H., Vilibić, I., Grčić, B., Ivatek-Šahdan, S., Tudor, M., and Djakovac, T.: Using multi-platform 4D-Var data assimilation to improve modeling of Adriatic Sea dynamics, Ocean Model., 146, 101538, https://doi.org/10.1016/j.ocemod.2019.101538, 2020.
Jansa, A., Monserrat, S., and Gomis, D.: The rissaga of 15 June 2006 in Ciutadella (Menorca), a meteorological tsunami, Adv. Geosci., 12, 1–4, https://doi.org/10.5194/adgeo-12-1-2007, 2007.
Jeong, J. and Hussain, F.: On the identification of a vortex, J. Fluid Mech., 285, 69–94, https://doi.org/10.1017/S0022112095000462, 1995.
Juza, M. and Tintoré, J.: Multivariate Sub-Regional Ocean Indicators in the Mediterranean Sea: From Event Detection to Climate Change Estimations, Front. Mar. Sci., 8, 233, https://doi.org/10.3389/fmars.2021.610589, 2021.
Juza, M., Mourre, B., Renault, L., Gómara, S., Sebastián, K., Lora, S., Beltran, J. P., Frontera, B., Garau, B., Troupin, C., Torner, M., Heslop, E., Casas, B., Escudier, R., Vizoso, G., and Tintoré, J.: SOCIB operational ocean forecasting system and multi-platform validation in the western mediterranean sea, J. Oper. Oceanogr., 9, 155–166, https://doi.org/10.1080/1755876X.2015.1117764, 2016.
Kaplan, D. M. and Lekien, F.: Spatial interpolation and filtering of surface current data based on open-boundary modal analysis, J. Geophys. Res.-Oceans, 112, 1–20, https://doi.org/10.1029/2006JC003984, 2007.
Kaplan, A., Kushnir, Y., Cane, M. A., and Blumenthal, M. B.: Reduced space optimal analysis for historical data sets: 136 years of Atlantic sea surface temperatures, J. Geophys. Res.-Oceans, 102, 27835–27860, https://doi.org/10.1029/97JC01734, 1997.
Kazeminezhad, M. H., Vilibić, I., Denamiel, C., Ghafarian, P., and Negah, S.: Weather radar and ancillary observations of the convective system causing the northern Persian Gulf meteotsunami on 19 March 2017, Nat. Hazards, 106, 1747–1769, https://doi.org/10.1007/s11069-020-04208-0, 2020.
Kim, S. Y., Terrill, E. J., and Cornuelle, B. D.: Mapping surface currents from HF radar radial velocity measurements using optimal interpolation, J. Geophys. Res.-Oceans, 113, 1–16, https://doi.org/10.1029/2007JC004244, 2008.
Kirincich, A.: Remote Sensing of the Surface Wind Field over the Coastal Ocean via Direct Calibration of HF Radar Backscatter Power, J. Atmos. Ocean. Technol., 33, 1377–1392, https://doi.org/10.1175/JTECH-D-15-0242.1, 2016a.
Kirincich, A.: The Occurrence, Drivers, and Implications of Submesoscale Eddies on the Martha's Vineyard Inner Shelf, J. Phys. Oceanogr., 46, 2645–2662, https://doi.org/10.1175/JPO-D-15-0191.1, 2016b.
Kohonen, T.: Self-organized formation of topologically correct feature maps, Biol. Cybern., 43, 59–69, https://doi.org/10.1007/BF00337288, 1982.
Kohonen, T.: Self-Organizing Maps, 3rd Edition., Springer-Verlag, Berlin, Heidelberg, New York, https://doi.org/10.1007/978-3-642-56927-2, 2001.
Kohonen, T., Kaski, S., Lagus, K., Salojärvi, J., Honkela, J., Paatero, V., and Saarela, A.: Self organization of a massive document collection, IEEE Trans. Neural Networks, 11, 574–585, 2000.
Kourafalou, V. H., De Mey, P., Staneva, J., Ayoub, N., Barth, A., Chao, Y., Cirano, M., Fiechter, J., Herzfeld, M., Kurapov, A., Moore, A. M., Oddo, P., Pullen, J., van der Westhuysen, A., and Weisberg, R. H.: Coastal Ocean Forecasting: science foundation and user benefits, J. Oper. Oceanogr., 8, 147–167, https://doi.org/10.1080/1755876X.2015.1022348, 2015a.
Kourafalou, V. H., De Mey, P., Staneva, J., Ayoub, N., Barth, A., Chao, Y., Cirano, M., Fiechter, J., Herzfeld, M., Kurapov, A., Moore, A. M., Oddo, P., Pullen, J., van der Westhuysen, A., and Weisberg, R. H.: Coastal Ocean Forecasting: science foundation and user benefits, J. Oper. Oceanogr., 8, 147–167, https://doi.org/10.1080/1755876X.2015.1022348, 2015b.
Lai, Y., Zhou, H., Yang, J., Zeng, Y., and Wen, B.: Submesoscale Eddies in the Taiwan Strait Observed by High-Frequency Radars: Detection Algorithms and Eddy Properties, J. Atmos. Ocean. Tech., 34, 939–953, https://doi.org/10.1175/JTECH-D-16-0160.1, 2017.
Lana, A., Marmain, J., Fernández, V., Tintoré, J., and Orfila, A.: Wind influence on surface current variability in the Ibiza Channel from HF Radar, Ocean Dynam., 66, 483–497, https://doi.org/10.1007/s10236-016-0929-z, 2016.
Laws, K. E., Vesecky, J. F., Lovellette, M. N., and Paduan, J. D.: Ship tracking by HF radar in coastal waters, in OCEANS 2016 MTS/IEEE Monterey, 1–8, https://doi.org/10.1109/OCEANS.2016.7761012, 2016.
Le Vu, B., Stegner, A., and Arsouze, T.: Angular Momentum Eddy Detection and Tracking Algorithm (AMEDA) and Its Application to Coastal Eddy Formation, J. Atmos. Ocean. Technol., 35, 739–762, https://doi.org/10.1175/JTECH-D-17-0010.1, 2018.
Lehahn, Y., d'Ovidio, F., Lévy, M., and Heifetz, E.: Stirring of the northeast Atlantic spring bloom: A Lagrangian analysis based on multisatellite data, J. Geophys. Res.-Oceans, 112, C08005, https://doi.org/10.1029/2006JC003927, 2007.
Lekien, F., Coulliette, C., Bank, R., and Marsden, J.: Open-boundary modal analysis: Interpolation, extrapolation, and filtering, J. Geophys. Res.-Oceans, 109, 1–13, https://doi.org/10.1029/2004JC002323, 2004.
Lekien, F., Coulliette, C., Mariano, A. J., Ryan, E. H., Shay, L. K., Haller, G., and Marsden, J.: Pollution release tied to invariant manifolds: A case study for the coast of Florida, Phys. D Nonlinear Phenom., 210, 1–20, https://doi.org/10.1016/j.physd.2005.06.023, 2005.
Ličer, M., Mourre, B., Troupin, C., Krietemeyer, A., Jansá, A., and Tintoré, J.: Numerical study of Balearic meteotsunami generation and propagation under synthetic gravity wave forcing, Ocean Model., 111, 38–45, https://doi.org/10.1016/j.ocemod.2017.02.001, 2017.
Ličer, M., Estival, S., Reyes-Suarez, C., Deponte, D., and Fettich, A.: Lagrangian modelling of a person lost at sea during the Adriatic scirocco storm of 29 October 2018, Nat. Hazards Earth Syst. Sci., 20, 2335–2349, https://doi.org/10.5194/nhess-20-2335-2020, 2020.
Lipa, B. and Nyden, B.: Directional wave information from the SeaSonde, IEEE J. Ocean. Eng., 30, 221–231, 2005.
Lipa, B., Barrick, D. E., and Maresca, J. W.: HF radar measurements of long ocean waves, J. Geophys. Res., 86, 4089–4102, 1981.
Lipa, B. J., Barrick, D. E., Isaacson, J., and Lilleboe, P. M.: CODAR wave measurements from a North Sea semisubmersible, IEEE J. Ocean. Eng., 15, 119–125, https://doi.org/10.1109/48.50697, 1990.
Lipa, B. J., Barrick, D. E., Bourg, J., and Nyden, B. B.: HF radar detection of tsunamis, J. Oceanogr., 62, 705–716, https://doi.org/10.1007/s10872-006-0088-9, 2006.
Lipa, B., Barrick, D., Saitoh, S.-I., Ishikawa, Y., Awaji, T., Largier, J., and Garfield, N.: Japan Tsunami Current Flows Observed by HF Radars on Two Continents, Remote Sens., 3, 1663–1679, https://doi.org/10.3390/rs3081663, 2011.
Lipa, B., Isaacson, J., Nyden, B., and Barrick, D.: Lipa, B. et al. Tsunami Arrival Detection with High Frequency (HF) Radar, Remote Sens., 4, 1448–1461, https://doi.org/10.3390/rs4113363, 2012.
Lipa, B., Barrick, D., and Isaacson, J.: Evaluating HF Coastal Radar Site Performance for Tsunami Warning, Remote Sens., 11, 2773, https://doi.org/10.3390/rs11232773, 2019.
Liu, Y. and Weisberg, R. H.: Evaluation of trajectory modeling in different dynamic regions using normalized cumulative Lagrangian separation, J. Geophys. Res.-Oceans, 116, 1–13, https://doi.org/10.1029/2010JC006837, 2011.
Liu, Y., Weisberg, R. H., and Mooers, C. N. K.: Performance evaluation of the self-organizing map for feature extraction, J. Geophys. Res.-Oceans, 111, 1–14, https://doi.org/10.1029/2005JC003117, 2006.
Liu, Y., Dong, C., Guan, Y., Chen, D., McWilliams, J., and Nencioli, F.: Eddy Analysis in the Subtropical Zonal Band of the North Pacific Ocean, Deep Sea Res. Pt. I, 68, 54–67, https://doi.org/10.1016/j.dsr.2012.06.001, 2012.
Long, A. E. and Trizna, D. B.: Measurements and preliminary interpretation of HF radar Doppler spectra from the sea echo of an Atlantic storm, Naval Research Laboratory: Washinghton, DC, USA, p. 7456, 1972.
Long, R., Barrick, D., Largier, J. and Garfield, N.: Wave Observations from Central California: SeaSonde Systems and In Situ Wave Buoys, J. Sensors, 2011, 1–18, https://doi.org/10.1155/2011/728936, 2011.
Lorente, P., Piedracoba, S., Soto-Navarro, J., and Alvarez-Fanjul, E.: Evaluating the surface circulation in the Ebro delta (northeastern Spain) with quality-controlled high-frequency radar measurements, Ocean Sci., 11, 921–935, https://doi.org/10.5194/os-11-921-2015, 2015.
Lorente, P., Piedracoba, S., Sotillo, M. G., Aznar, R., Amo-Balandron, A., Pascual, A., Soto-Navarro, J., and Álvarez-Fanjul, E.: Characterizing the surface circulation in Ebro Delta (NW Mediterranean) with HF radar and modeled current data, J. Mar. Syst., 163, 61–79, https://doi.org/10.1016/j.jmarsys.2016.07.001, 2016.
Lorente, P., García-Sotillo, M., Amo-Baladrón, A., Aznar, R., Levier, B., Sánchez-Garrido, J. C., Sammartino, S., de Pascual-Collar, Á., Reffray, G., Toledano, C., and Álvarez-Fanjul, E.: Skill assessment of global, regional, and coastal circulation forecast models: evaluating the benefits of dynamical downscaling in IBI (Iberia–Biscay–Ireland) surface waters, Ocean Sci., 15, 967–996, https://doi.org/10.5194/os-15-967-2019, 2019a.
Lorente, P., Piedracoba, S., Sotillo, M. G., and álvarez-Fanjul, E.: Long-term monitoring of the Atlantic jet through the strait of gibraltar with HF radar observations, J. Mar. Sci. Eng., 7, https://doi.org/10.3390/jmse7010003, 2019b.
Lorente, P., Sotillo, M. G., Amo-Baladrón, A., Aznar, R., Levier, B., Aouf, L., Dabrowski, T., Pascual, Á. De, Reffray, G., Dalphinet, A., Toledano, C., Rainaud, R., and Álvarez-Fanjul, E.: The NARVAL Software Toolbox in Support of Ocean Models Skill Assessment at Regional and Coastal Scales, in ICCS 2019, Lecture Notes in Computer Science, edited by: Rodrigues, J., (Cham: Springer), https://doi.org/10.1007/978-3-030-22747-0_25, 2019c.
Lorente, P., Lin-Ye, J., García-León, M., Reyes, E., Fernandes, M., Sotillo, M. G., Espino, M., Ruiz, M. I., Gracia, V., Perez, S., Aznar, R., Alonso-Martirena, A., and Álvarez-Fanjul, E.: On the Performance of High Frequency Radar in the Western Mediterranean During the Record-Breaking Storm Gloria, Front. Mar. Sci., 8, 205, https://doi.org/10.3389/fmars.2021.645762, 2021.
Lorente, P., Aguiar, E., Bendoni, M., Berta, M., Brandini, C., Cáceres-Euse, A., Capodici, F., Cianelli, D., Ciraolo, G., Corgnati, L., Dadić, V., Doronzo, B., Drago, A., Dumas, D., Falco, P., Fattorini, M., Gauci, A., Gómez, R., Griffa, A., Guérin, C.-A., Hernández-Carrasco, I., Hernández-Lasheras, J., Ličer, M., Magaldi, M. G., Mantovani, C., Mihanovic, H., Molcard, A., Mourre, B., Orfila, A., Révelard, A., Reyes, E., Sanchez, J., Saviano, S., Sciascia, R., Taddei, S., Tintoré, J., Toledo, Y., Ursella, L., Uttieri, M., Vilibić, I., Zambianchi, E., and Cardín, V.: Coastal high-frequency radars in the Mediterranean – Part 1: Status of operations and a framework for future development, Ocean Sci., 18, 761–795, https://doi.org/10.5194/os-18-761-2022, 2022.
Mandal, S., Sil, S., Pramanik, S., Arunraj, K. S., and Jena, B. K.: Characteristics and evolution of a coastal mesoscale eddy in the Western Bay of Bengal monitored by high-frequency radars, Dyn. Atmos. Ocean., 88, 101107, https://doi.org/10.1016/j.dynatmoce.2019.101107, 2019.
Manso-Narvarte, I., Caballero, A., Rubio, A., Dufau, C., and Birol, F.: Joint analysis of coastal altimetry and high-frequency (HF) radar data: observability of seasonal and mesoscale ocean dynamics in the Bay of Biscay, Ocean Sci., 14, 1265–1281, https://doi.org/10.5194/os-14-1265-2018, 2018.
Manso-Narvarte, I., Fredj, E., Jordà, G., Berta, M., Griffa, A., Caballero, A., and Rubio, A.: 3D reconstruction of ocean velocity from high-frequency radar and acoustic Doppler current profiler: a model-based assessment study, Ocean Sci., 16, 575–591, https://doi.org/10.5194/os-16-575-2020, 2020.
Manso-Narvarte, I., Rubio, A., Jordà, G., Carpenter, J., Merckelbach, L., and Caballero, A.: Three-Dimensional Characterization of a Coastal Mode-Water Eddy from Multiplatform Observations and a Data Reconstruction Method, Remote Sens., 13, 674, https://doi.org/10.3390/rs13040674, 2021.
Mantovani, C., Corgnati, L., Horstmann, J., Rubio, A., Reyes, E., Quentin, C., Cosoli, S., Asensio, J. L., Mader, J., and Griffa, A.: Best Practices on High Frequency Radar Deployment and Operation for Ocean Current Measurement, Front. Mar. Sci., 7, 210, https://doi.org/10.3389/fmars.2020.00210, 2020.
March, D., Metcalfe, K., Tintoré, J., and Godley, B. J.: Tracking the global reduction of marine traffic during the COVID-19 pandemic, Nat. Commun., 12, 2415, https://doi.org/10.1038/s41467-021-22423-6, 2021.
Maresca, S., Braca, P., Horstmann, J., and Grasso, R.: Maritime Surveillance Using Multiple High-Frequency Surface-Wave Radars, IEEE Trans. Geosci. Remote Sens., 52, 5056–5071, https://doi.org/10.1109/TGRS.2013.2286741, 2013.
Marmain, J., Molcard, A., Forget, P., Barth, A., and Ourmières, Y.: Assimilation of HF radar surface currents to optimize forcing in the northwestern Mediterranean Sea, Nonlin. Processes Geophys., 21, 659–675, https://doi.org/10.5194/npg-21-659-2014, 2014.
McWilliams, J. C.: A survey of submesoscale currents, Geosci. Lett., 6, 3, https://doi.org/10.1186/s40562-019-0133-3, 2019.
Meadows, L., Whelan, C., Barrick, D., Kroodsma, R., Ruf, C., Teague, C., Meadows, G., and Wang, S.: High frequency radar and its application to fresh water, J. Great Lakes Res., 39, 183–193, https://doi.org/10.1016/j.jglr.2013.01.002, 2013.
Medail, F. and Queìzel, P.: Hot-Spots Analysis for Conservation of Plant Biodiversity in the Mediterranean Basin, Ann. Missouri Bot. Gard., 84, 112–127, https://doi.org/10.2307/2399957, 1997.
Menemenlis, D., Fukumori, I., and Lee, T.: Atlantic to Mediterranean Sea Level Difference Driven by Winds near Gibraltar Strait, J. Phys. Oceanogr., 37, 359–376, https://doi.org/10.1175/JPO3015.1, 2007.
Meola, B., Webster, C., Agardi, T., Bernal, M., Borg, J. A., Calò, A., Cebrian, D., Cebrian, D., Claudet, J., Daméry, C., David, L., Davis, J., El Asmi, S., Giakoumi, S., Gomei, M., Guidetti, P., Hoyt, E., Grissac, A., Kizilkaya, Z., and Tunesi, L.: The 2016 status of Marine Protected Areas in the Mediterranean, MedPAN & RAC/SPA., MedPAN Collection, https://www.um.edu.mt/library/oar/handle/123456789/69286 (last access: 19 May 2022), 2019.
Miles, T., Seroka, G., and Glenn, S.: Coastal ocean circulation during Hurricane Sandy, J. Geophys. Res.-Oceans, 122, 7095–7114, https://doi.org/10.1002/2017JC013031, 2017.
Millot, C.: Circulation in the Western Mediterranean Sea, J. Mar. Syst., 20, 423–442, https://doi.org/10.1016/S0924-7963(98)00078-5, 1999.
Mitchell, J., Lowe, J., Wood, R., and Vellinga, M.: Extreme events due to human-induced climate change, Philos. T. Roy. Soc. A, 364, 2117–2133, https://doi.org/10.1098/rsta.2006.1816, 2006.
Mkhinini, N., Coimbra, A. L. S., Stegner, A., Arsouze, T., Taupier-Letage, I., and Béranger, K.: Long-lived mesoscale eddies in the eastern Mediterranean Sea: Analysis of 20 years of AVISO geostrophic velocities, J. Geophys. Res.-Oceans, 119, 8603–8626, https://doi.org/10.1002/2014JC010176, 2014.
Molcard, A., Poulain, P. M., Forget, P., Griffa, A., Barbin, Y., Gaggelli, J., De Maistre, J. C., and Rixen, M.: Comparison between VHF radar observations and data from drifter clusters in the Gulf of La Spezia (Mediterranean Sea), J. Mar. Syst., 78, 79–89, https://doi.org/10.1016/j.jmarsys.2009.01.012, 2009.
Monserrat, S., Vilibić, I., and Rabinovich, A. B.: Meteotsunamis: atmospherically induced destructive ocean waves in the tsunami frequency band, Nat. Hazards Earth Syst. Sci., 6, 1035–1051, https://doi.org/10.5194/nhess-6-1035-2006, 2006.
Moore, A. M., Martin, M. J., Akella, S., Arango, H. G., Balmaseda, M., Bertino, L., Ciavatta, S., Cornuelle, B., Cummings, J., Frolov, S., Lermusiaux, P., Oddo, P., Oke, P. R., Storto, A., Teruzzi, A., Vidard, A., and Weaver, A. T.: Synthesis of Ocean Observations Using Data Assimilation for Operational, Real-Time and Reanalysis Systems: A More Complete Picture of the State of the Ocean, Front. Mar. Sci., 6, 90, https://doi.org/10.3389/fmars.2019.00090, 2019.
Morrow, R., Birol, F., Griffin, D., and Sudre, J.: Divergent pathways of cyclonic and anti-cyclonic ocean eddies, Geophys. Res. Lett., 31, https://doi.org/10.1029/2004GL020974, 2004.
Mourre, B., Aguiar, E., Juza, M., Hernandez-Lasheras, J., Reyes, E., Heslop, E., Escudier, R., Cutolo, E., Ruiz, S., Mason, E., Pascual, A., and Tintoré, J.: Assessment of High-Resolution Regional Ocean Prediction Systems Using Multi-Platform Observations: Illustrations in the Western Mediterranean Sea, in: New Frontiers in Operational Oceanography, edited by: Chassignet, E., Pascual, A., Tintoré, J., and Verron, J., 663–694, GODAE Ocean View, https://doi.org/10.17125/gov2018.ch24, 2018.
Mourre, B., Santana, A., Buils, A., Gautreau, L., Ličer, M., Jansà, A., Casas, B., Amengual, B., and Tintoré, J.: On the potential of ensemble forecasting for the prediction of meteotsunamis in the Balearic Islands: sensitivity to atmospheric model parameterizations, Nat. Hazards, 106, 1315–1336, https://doi.org/10.1007/s11069-020-03908-x, 2020.
Mundaca-Moraga, V., Abarca-del-Rio, R., Figueroa, D., and Morales, J.: A Preliminary Study of Wave Energy Resource Using an HF Marine Radar, Application to an Eastern Southern Pacific Location: Advantages and Opportunities, Remote Sens., 13, 203, https://doi.org/10.3390/rs13020203, 2021.
Nencioli, F., Dong, C., Dickey, T., Washburn, L., and McWilliams, J. C.: A Vector Geometry- Based Eddy Detection Algorithm and Its Application to a High-Resolution Numerical Model Product and High-Frequency Radar Surface Velocities in the Southern California Bight, J. Atmos. Ocean. Technol., 27, 564–579, https://doi.org/10.1175/2009JTECHO725.1, 2010.
Nishimoto, M. M. and Washburn, L.: Patterns of coastal eddy circulation and abundance of pelagic juvenile fish in the Santa Barbara Channel, California, USA, Mar. Ecol. Prog. Ser., 241, 183–199, 2002.
Oke, P. R., Allen, J. S., Miller, R. N., Egbert, G. D., and Kosro, P. M.: Assimilation of surface velocity data into a primitive equation coastal ocean model, J. Geophys. Res.-Oceans, 107, 5–25, https://doi.org/10.1029/2000JC000511, 2002.
Okubo, A.: Horizontal dispersion of floatable particles in the vicinity of velocity singularities such as convergences, Deep Sea Res., 17, 445–454, https://doi.org/10.1016/0011-7471(70)90059-8, 1970.
Orasi, A., Picone, M., Drago, A., Capodici, F., Gauci, A., Nardone, G., Inghilesi, R., Azzopardi, J., Galea, A., Ciraolo, G., Sánchez Musulin, J., and Alonso-Martirena, A.: HF radar for wind waves measurements in the Malta-Sicily Channel, Meas. J. Int. Meas. Confed., 128, 446–454, https://doi.org/10.1016/j.measurement.2018.06.060, 2018.
Orfila, A., Balle, S., and Simarro, G.: Topographic enhancement of long waves generated by an idealized moving pressure system, Sci. Mar., 75, 595–603, https://doi.org/10.3989/scimar.2011.75n3595, 2011.
Orfila, A., Molcard, A., Sayol, J. M., Marmain, J., Bellomo, L., Quentin, C., and Barbin, Y.: Empirical forecasting of HF-radar velocity using genetic algorithms, IEEE Trans. Geosci. Remote Sens., 53, 2875–2886, https://doi.org/10.1109/TGRS.2014.2366294, 2015.
Orlić, M.: The first attempt at cataloguing tsunami-like waves of meteorological origin in Croatian coastal waters, Acta Adriat. Int. J. Mar. Sci., 56, 83–95, 2015.
Paduan, J. D. and Graber, H. C.: Introduction to high-frequency radar: Reality and myth, Oceanography, 10, 36–39, https://doi.org/10.5670/oceanog.1997.18, 1997.
Paduan, J. D. and Shulman, I.: HF radar data assimilation in the Monterey Bay area, J. Geophys. Res.-Oceans, 109, 1–17, https://doi.org/10.1029/2003JC001949, 2004.
Paduan, J. D. and Washburn, L.: High-frequency radar observations of ocean surface currents, Ann. Rev. Mar. Sci., 5, 115–136, https://doi.org/10.1146/annurev-marine-121211-172315, 2013.
Papadopoulos, G. A.: On some exceptional seismic (?) sea-waves inthe Greek archipelago, Sci. Tsunami Hazards, 11, 25–34, 1993.
Péliz, Á., Teles-Machado, A., Marchesiello, P., Dubert, J., and Lafuente, J.: Filament generation off the Strait of Gibraltar in response to Gap winds, Dyn. Atmos. Ocean., 46, 36–45, https://doi.org/10.1016/j.dynatmoce.2008.08.002, 2009.
Piedracoba, S., Rosón, G., and Varela, R. A.: Origin and development of recurrent dipolar vorticity structures in the outer Ría de Vigo (NW Spain), Cont. Shelf Res., 118, 143–153, https://doi.org/10.1016/j.csr.2016.03.001, 2016.
Ponsford, A. M., Sevgi, L., and Chan, H.: An integrated maritime surveillance system based on high-frequency surface-wave radars, 2. Operational status and system performance, Antennas Propag. Mag. IEEE, 43, 52–63, https://doi.org/10.1109/74.979367, 2001.
Poulain, P.-M., Mauri, E., Gerin, R., Chiggiato, J., Schroeder, K., Griffa, A., Borghini, M., Zambianchi, E., Falco, P., Testor, P., and Mortier, L.: On the dynamics in the southeastern Ligurian Sea in summer 2010, Cont. Shelf Res., 196, 104083, https://doi.org/10.1016/j.csr.2020.104083, 2020.
Preisendorfer, R. W. and Mobley, C. D.: Principal component analysis in meteorology and oceanography, Amsterdam, New York, Elsevier , New York, NY, USA, Distributors for the U.S. and Canada, Elsevier Science Pub. Co., 1988.
Ren, L., Hu, Z., and Hartnett, M.: Short-Term Forecasting of Coastal Surface Currents Using High Frequency Radar Data and Artificial Neural Networks, Remote Sens., 10, 850, https://doi.org/10.3390/rs10060850, 2018.
Révelard, A., Reyes, E., Mourre, B., Hernández-Carrasco, I., Rubio, A., Lorente, P., Fernández, C. D. L., Mader, J., Álvarez-Fanjul, E., and Tintoré, J.: Sensitivity of Skill Score Metric to Validate Lagrangian Simulations in Coastal Areas: Recommendations for Search and Rescue Applications, Front. Mar. Sci., 8, 630388, https://doi.org/10.3389/fmars.2021.630388, 2021.
Reyes, E.: A high-resolution modeling study of the ocean response to wind forcing within the Strait of Gibraltar, 27 February, PhD Thesis, 230 pp., https://doi.org/10.13140/RG.2.2.28881.07525, 2015.
Reyes, E., Rotllán-García, P., Rubio, A., Corgnati, L., Mader, J., and Mantovani, C.: Guidelines on how to sync your High Frequency (HF) radar data with the European HF Radar node (Version 1.2), Balearic Islands Coastal Observing and Forecasting System, SOCIB, https://doi.org/10.25704/9XPF-76G7, 2019.
Reyes, E., Hernández-Carrasco, I., Révelard, A., Mourre, B., Rotllán, P., Comerma, E., Bakhsh, T.T., Rubio, A., Mader, J., Ferrer, L., De Lera Fernández, C., Álvarez-Fanjul, E., and Tintoré, J.: IBISAR service for real-time data ranking in the IBI area for emergency responders and SAR operators, in: Copernicus Marine Service Ocean State Report, Issue 4, J. Oper. Oceanogr., 13, 92–99, https://doi.org/10.1080/1755876X.2020.1785097, 2020a.
Reyes, E., Mourre, B., Hernández-Lasheras, J., Hernández-Carrasco, I., Wirth, N., Balaguer, P., Casas, B., and Tintoré, J. SOCIB INT RadarAPM Nov2018. Lagrangian Experiment Ibiza Channel (Version [x.y.z]) [Data set], Balearic Islands Coastal Observing and Forecasting System, SOCIB. https://doi.org/10.25704/84ZE-SF42, 2020b.
Reyes, E., Mourre, B., Wirth, N., Balaguer, P., Casas, B., Troupin, C., Muñoz, C., Orfila, A., and Tintoré, J. SOCIB INT RadarAPM Jul2016. Lagrangian Experiment Ibiza Channel (Version [1.0.0]) [Data set], Balearic Islands Coastal Observing and Forecasting System, SOCIB. https://doi.org/10.25704/BB7M-ZV61, 2020c.
Rinaldi, E., Buongiorno Nardelli, B., Zambianchi, E., Santoleri, R., and Poulain, P.-M.: Lagrangian and Eulerian observations of the surface circulation in the Tyrrhenian Sea, J. Geophys. Res.-Oceans, 115, C04024, https://doi.org/10.1029/2009JC005535, 2010.
Roarty, H., Glenn, S., Kohut, J., Gong, D., Handel, E., Rivera, E., Garner, T., Atkinson, L., Brown, W., Jakubiak, C., Muglia, M., Haines, S., and Seim, H.: Operation and Application of a Regional High-Frequency Radar Network in the Mid-Atlantic Bight, Mar. Technol. Soc. J., 44, 133–145, 2010.
Roarty, H., Cook, T., Hazard, L., Harlan, J., Cosoli, S., Wyatt, L., Fanjul, E. A., Terrill, E., Otero, M., Largier, J., Glenn, S., Ebuchi, N., Whitehouse, B., Bartlett, K., Mader, J., Rubio, A., Corgnati, L. P., Mantovani, C., Griffa, A., Reyes, E., Lorente, P., Flores-Vidal, X., Rogowski, P., Prukpitikul, S., Lee, S. H., Lai, J. W., Guerin, C., Sanchez, J., Hansen, B., Grilli, S., and Matta, K. S.: The global high frequency radar network, Front. Mar. Sci., 6, 164, https://doi.org/10.3389/fmars.2019.00164, 2019.
Robinson, A. R.: Overview and Summary of Eddy Science BT – Eddies in Marine Science, edited by: Robinson, A. R., Springer Berlin Heidelberg, Berlin, Heidelberg, 3–15, 1983.
Robinson, I.: Discovering the Ocean from Space, Discov. Ocean from Sp. by Ian S. Robinson, Berlin Springer, 2010, ISBN 978-3-540-24430-1, https://doi.org/10.1007/978-3-540-68322-3_1, 2010.
Romero, R., Vich, M.-M., and Ramis, C.: A pragmatic approach for the numerical prediction of meteotsunamis in Ciutadella harbour (Balearic Islands), Ocean Model., 142, 101441, https://doi.org/10.1016/j.ocemod.2019.101441, 2019.
Rubio, A., Mader, J., Corgnati, L., Mantovani, C., Griffa, A., Novellino, A., Quentin, C., Wyatt, L., Schulz-Stellenfleth, J., Horstmann, J., Lorente, P., Zambianchi, E., Hartnett, M., Fernandes, C., Zervakis, V., Gorringe, P., Melet, A., and Puillat, I.: HF Radar activity in European coastal seas: Next steps toward a Pan-European HF Radar network, Front. Mar. Sci., 4, 1–17, https://doi.org/10.3389/fmars.2017.00008, 2017.
Rubio, A., Caballero, A., Orfila, A., Hernández-Carrasco, I., Ferrer, L., González, M., Solabarrieta, L., and Mader, J.: Eddy-induced cross-shelf export of high Chl a coastal waters in the SE Bay of Biscay, Remote Sens. Environ., 205, 290–304, https://doi.org/10.1016/j.rse.2017.10.037, 2018.
Rubio, A., Hernández-Carrasco, I., Orfila, A., González, M., Reyes, E., Corgnati, L., Berta, M., Griffa, A., and Mader, J.: A Lagrangian approach to monitor local particle retention conditions in coastal areas, in: Copernicus Marine Service Ocean State Report, Issue 4, J. Oper. Oceanogr., 13, 54–59, https://doi.org/10.1080/1755876X.2020.1785097, 2020.
Rubio, A., Reyes, E., Mantovani, C., Corgnati, L., Lorente, P., Solabarrieta, L., Mader, J., Fernández, V., Pouliquen, S., Novellino, A., Karstensen, J., and Petihakis, G.: European High Frequency Radar network governance, D3.4, EuroSea Deliverable, 41 pp., https://doi.org/10.3289/eurosea_d3.4, 2021.
Ruiz-Parrado, I., Genua-Olmedo, A., Reyes, E., Mourre, B., Rotllán, P., Lorente, P., García-Sotillo, M., and Tintoré, J.: Coastal ocean variability related to the most extreme Ebro River discharge over the last 15 years, in: Copernicus Marine Service Ocean State Report, Issue 4, J. Oper. Oceanogr., 13:sup1, 160–165, https://doi.org/10.1080/1755876X.2020.1785097, 2020.
Ryabinin, V., Barbière, J., Haugan, P., Kullenberg, G., Smith, N., McLean, C., Troisi, A., Fischer, A., Aricò, S., Aarup, T., Pissierssens, P., Visbeck, M., Enevoldsen, H. O. and Rigaud, J.: The UN Decade of Ocean Science for Sustainable Development, Front. Mar. Sci., 6, 460, https://doi.org/10.3389/fmars.2019.00470, 2019.
Ryan, P. G., Moore, C. J., van Franeker, J. A., and Moloney, C. L.: Monitoring the abundance of plastic debris in the marine environment, Philos. T. Roy. Soc. B, 364, 1999–2012, https://doi.org/10.1098/rstb.2008.0207, 2009.
Sadarjoen, I. A., Post, F. H., Ma, B., Banks, D. C., and Pagendarm, H.-G.: Selective visualization of vortices in hydrodynamic flows, in: Proceedings Visualization'98 (Cat. No.98CB36276), 419–422, 1998.
Sahal, A., Roger, J., Allgeyer, S., Lemaire, B., Hébert, H., Schindelé, F., and Lavigne, F.: The tsunami triggered by the 21 May 2003 Boumerdès-Zemmouri (Algeria) earthquake: field investigations on the French Mediterranean coast and tsunami modelling, Nat. Hazards Earth Syst. Sci., 9, 1823–1834, https://doi.org/10.5194/nhess-9-1823-2009, 2009.
Sánchez-Arcilla, A., García-León, M., and Gracia, V.: Hydro-morphodynamic modelling in Mediterranean storms – errors and uncertainties under sharp gradients, Nat. Hazards Earth Syst. Sci., 14, 2993–3004, https://doi.org/10.5194/nhess-14-2993-2014, 2014.
Sánchez-Garrido, J. C., García Lafuente, J., Álvarez Fanjul, E., Sotillo, M. G., and de los Santos, F. J.: What does cause the collapse of the Western Alboran Gyre? Results of an operational ocean model, Prog. Oceanogr., 116, 142–153, https://doi.org/10.1016/j.pocean.2013.07.002, 2013.
Saviano, S., Kalampokis, A., Zambianchi, E., and Uttieri, M.: A year-long assessment of wave measurements retrieved from an HF radar network in the Gulf of Naples (Tyrrhenian Sea, Western Mediterranean Sea), J. Oper. Oceanogr., 12, 1–15, https://doi.org/10.1080/1755876X.2019.1565853, 2019.
Saviano, S., Cianelli, D., Zambianchi, E., Conversano, F., and Uttieri, M.: An Integrated Reconstruction of the Multiannual Wave Pattern in the Gulf of Naples (South-Eastern Tyrrhenian Sea, Western Mediterranean Sea), J. Mar. Sci. Eng., 8, 372, https://doi.org/10.3390/jmse8050372, 2020.
Saviano, S., Esposito, G., Di Lemma, R., de Ruggiero, P., Zambianchi, E., Pierini, S., Falco, P., Buonocore, B., Cianelli, D., and Uttieri, M.: Wind Direction Data from a Coastal HF Radar System in the Gulf of Naples (Central Mediterranean Sea), Remote Sens., 13, 1333, https://doi.org/10.3390/rs13071333, 2021.
Saviano, S., Biancardi, A. A., Uttieri, M., Zambianchi, E., Cusati, L. A., Pedroncini, A., Contento, G., and Cianelli, D.: Sea Storm Analysis: Evaluation of Multiannual Wave Parameters Retrieved from HF Radar and Wave Model, Remote Sens., 14, 1696, https://doi.org/10.3390/rs14071696, 2022.
Sayol, J. M., Orfila, A., Simarro, G., Conti, D., Renault, L., and Molcard, A.: A Lagrangian model for tracking surface spills and SaR operations in the ocean, Environ. Model. Softw., 52, 74–82, https://doi.org/10.1016/j.envsoft.2013.10.013, 2014.
Schaeffer, A., Molcard, A., Forget, P., Fraunié, P., and Garreau, P.: Generation mechanisms for mesoscale eddies in the Gulf of Lions: radar observation and modeling, Ocean Dynam., 61, 1587–1609, https://doi.org/10.1007/s10236-011-0482-8, 2011.
Sciascia, R., Berta, M., Carlson, D. F., Griffa, A., Panfili, M., La Mesa, M., Corgnati, L., Mantovani, C., Domenella, E., Fredj, E., Magaldi, M. G., D'Adamo, R., Pazienza, G., Zambianchi, E., and Poulain, P.-M.: Linking sardine recruitment in coastal areas to ocean currents using surface drifters and HF radar: a case study in the Gulf of Manfredonia, Adriatic Sea, Ocean Sci., 14, 1461–1482, https://doi.org/10.5194/os-14-1461-2018, 2018.
Šepić, J., Vilibić, I., and Belušić, D.: Source of the 2007 Ist meteotsunami (Adriatic Sea), J. Geophys. Res.-Oceans, 114, C03016, https://doi.org/10.1029/2008JC005092, 2009.
Šepić, J., Vilibić, I., Rabinovich, A. B., and Monserrat, S.: Widespread tsunami-like waves of 23–27 June in the Mediterranean and Black Seas generated by high-altitude atmospheric forcing, Sci. Rep., 5, 11682, https://doi.org/10.1038/srep11682, 2015.
Shay, L. K., Graber, H. C., Ross, D. B., and Chapman, R. D.: Mesoscale Ocean Surface Current Structure Detected by High-Frequency Radar, J. Atmos. Ocean. Tech., 12, 881–900, https://doi.org/10.1175/1520-0426(1995)012< 0881:MOSCSD>2.0.CO;2, 1995.
Shay, L., Cook, T., Haus, B., Martinez-Pedraja, J., Peters, H., Mariano, A., VanLeer, J., An, P., Smith, S., Soloviev, A., Weisberg, R., and Luther, M.: VHF radar detects oceanic submesoscale vortex along Florida Coast, EOS Trans., 81, 209–213, https://doi.org/10.1029/00EO00143, 2000.
Shchepetkin, A. F. and McWilliams, J. C.: A method for computing horizontal pressure-gradient force in an oceanic model with a nonaligned vertical coordinate, J. Geophys. Res.-Oceans, 108, 3090, https://doi.org/10.1029/2001JC001047, 2003.
Shchepetkin, A. F. and McWilliams, J. C.: The regional oceanic modeling system (ROMS): a split-explicit, free-surface, topography-following-coordinate oceanic model, Ocean Model., 9, 347–404, https://doi.org/10.1016/j.ocemod.2004.08.002, 2005.
Shen, W., Gurgel, K.-W., Voulgaris, G., Schlick, T., and Stammer, D.: Wind-speed inversion from HF radar first-order backscatter signal, Ocean Dynam., 62, 105–121, https://doi.org/10.1007/s10236-011-0465-9, 2012.
Shen, W. and Gurgel, K. W.: Wind direction inversion from narrow-beam HF radar backscatter signals in low and high wind conditions at different radar frequencies, Remote Sens., 10, 1480, https://doi.org/10.3390/rs10091480, 2018.
Shrira, V. I. and Forget, P.: On the Nature of Near-Inertial Oscillations in the Uppermost Part of the Ocean and a Possible Route toward HF Radar Probing of Stratification, J. Phys. Oceanogr., 45, 2660–2678, https://doi.org/10.1175/JPO-D-14-0247.1, 2015.
Sikora, A.: European Green Deal – legal and financial challenges of the climate change, ERA Forum, 21, 681–697, https://doi.org/10.1007/s12027-020-00637-3, 2021.
Solabarrietaa, L., Frolov, S., Cook, M., Paduan, J., Rubio, A., González, M., Mader, J., and Charria, G.: Skill assessment of HF radar-derived products for Lagrangian simulations in the Bay Of Biscay, J. Atmos. Ocean. Tech., 33, 2585–2597, https://doi.org/10.1175/JTECH-D-16-0045.1, 2016.
Solabarrieta, L., Hernández-Carrasco, I., Rubio, A., Campbell, M., Esnaola, G., Mader, J., Jones, B. H., and Orfila, A.: A new Lagrangian-based short-term prediction methodology for high-frequency (HF) radar currents, Ocean Sci., 17, 755–768, https://doi.org/10.5194/os-17-755-2021, 2021.
Sotillo, M. G., Cailleau, S., Lorente, P., Levier, B., Aznar, R., Reffray, G., Amo-Baladrón, A., Chanut, J., Benkiran, M., and Álvarez-Fanjul, E.: The MyOcean IBI ocean forecast and reanalysis systems: Operational products and roadmap to the future copernicus service, J. Oper. Oceanogr., 8, 63–79, https://doi.org/10.1080/1755876X.2015.1014663, 2015.
Sotillo, M. G., Mourre, B., Mestres, M., Lorente, P., Aznar, R., García-León, M., Liste, M., Santana, A., Espino, M., and Álvarez, E.: Evaluation of the Operational CMEMS and Coastal Downstream Ocean Forecasting Services During the Storm Gloria (January 2020), Front. Mar. Sci., 8, 300, https://doi.org/10.3389/fmars.2021.644525, 2021.
Soto-Navarro, J., Jordá, G., Compa, M., Alomar, C., Fossi, M. C., and Deudero, S.: Impact of the marine litter pollution on the Mediterranean biodiversity: A risk assessment study with focus on the marine protected areas, Mar. Pollut. Bull., 165, 112169, https://doi.org/10.1016/j.marpolbul.2021.112169, 2021.
Stott, P.: How climate change affects extreme weather events, Science, 352, 1517–1518, https://doi.org/10.1126/science.aaf7271, 2016.
Terrier, M., Pedreros, R., and Poisson, B.: Tsunamis: étude de cas au niveau de la côte méditerranéenne française – Rapport de synthèse, Rapport BRGM-RP-55765-Fr, 98 p, 31 fig, 7 tabl, 6 pl. h.L. http://infoterre.brgm.fr/rapports/RP-55765-FR.pdf (last access: 19 May 2022), 2007.
Tessier, E., Garnier, C., Mullot, J. ulrich, Lenoble, V., Arnaud, M., Raynaud, M., and Mounier, S.: Study of the spatial and historical distribution of sediment inorganic contamination in the Toulon Bay (France), Mar. Pollut. Bull., 62, 2075–2086, https://doi.org/10.1016/j.marpolbul.2011.07.022, 2011.
Tew Kai, E., Rossi, V., Sudre, J., Weimerskirch, H., Lopez, C., Hernandez-Garcia, E., Marsac, F., and Garçon, V.: Top marine predators track Lagrangian coherent structures, P. Natl. Acad. Sci. USA, 106, 8245–8250, https://doi.org/10.1073/pnas.0811034106, 2009.
Tintoré, J., Vizoso, G., Casas, B., Heslop, E., Pascual, A., Orfila, A., Ruiz, S., Martínez-Ledesma, M., Torner, M., Cusí, S., Diedrich, A., Balaguer, P., Gómez-Pujol, L., Álvarez-Ellacuria, A., Gómara, S., Sebastian, K., Lora, S., Beltrán, J. P., Renault, L., Juzà, M., Álvarez, D., March, D., Garau, B., Castilla, C., Cañellas, T., Roque, D., Lizarán, I., Pitarch, S., Carrasco, M. A., Lana, A., Mason, E., Escudier, R., Conti, D., Sayol, J. M., Barceló, B., Alemany, F., Reglero, P., Massuti, E., Vélez-Belchí, P., Ruiz, J., Oguz, T., Gómez, M., Álvarez, E., Ansorena, L., and Manriquez, M.: SOCIB: The Balearic islands coastal ocean observing and forecasting system responding to science, technology and society needs, Mar. Technol. Soc. J., 47, 101–117, https://doi.org/10.4031/MTSJ.47.1.10, 2013.
Tintoré, J., Lana, A., Marmain, J., Fernández, V., and Orfila, A.: SOCIB EXP RADAR Sep2014 (Version 1.0) [Data set], Brussels: Balearic Islands Coastal Observing and Forecasting System, SOCIB, https://doi.org/10.25704/MHBG-Q265, 2014.
Tintoré, J., Pinardi, N., Álvarez-Fanjul, E., Aguiar, E., Álvarez-Berastegui, D., Bajo, M., Balbin, R., Bozzano, R., Nardelli, B. B., Cardin, V., Casas, B., Charcos-Llorens, M., Chiggiato, J., Clementi, E., Coppini, G., Coppola, L., Cossarini, G., Deidun, A., Deudero, S., D'Ortenzio, F., Drago, A., Drudi, M., El Serafy, G., Escudier, R., Farcy, P., Federico, I., Fernández, J. G., Ferrarin, C., Fossi, C., Frangoulis, C., Galgani, F., Gana, S., García Lafuente, J., Sotillo, M. G., Garreau, P., Gertman, I., Gómez-Pujol, L., Grandi, A., Hayes, D., Hernández-Lasheras, J., Herut, B., Heslop, E., Hilmi, K., Juza, M., Kallos, G., Korres, G., Lecci, R., Lazzari, P., Lorente, P., Liubartseva, S., Louanchi, F., Malacic, V., Mannarini, G., March, D., Marullo, S., Mauri, E., Meszaros, L., Mourre, B., Mortier, L., Muñoz-Mas, C., Novellino, A., Obaton, D., Orfila, A., Pascual, A., Pensieri, S., Pérez Gómez, B., Pérez Rubio, S., Perivoliotis, L., Petihakis, G., de la Villéon, L. P., Pistoia, J., Poulain, P. M., Pouliquen, S., Prieto, L., Raimbault, P., Reglero, P., Reyes, E., Rotllan, P., Ruiz, S., Ruiz, J., Ruiz, I., Ruiz-Orejón, L. F., Salihoglu, B., Salon, S., Sammartino, S., Sánchez Arcilla, A., Sannino, G., Sannino, G., Santoleri, R., Sardá, R., Schroeder, K., Simoncelli, S., Sofianos, S., Sylaios, G., Tanhua, T., Teruzzi, A., Testor, P., Tezcan, D., Torner, M., et al.: Challenges for Sustained Observing and Forecasting Systems in the Mediterranean Sea, Front. Mar. Sci., 6, 568, https://doi.org/10.3389/fmars.2019.00568, 2019.
Tintoré, J., Lana, A., Marmain, J., Fernández, V., Casas, B., and Reyes, E.: HF Radar Ibiza data from date 2012-06-01 (Version 1.0.1) [Data set], Balearic Islands Coastal Observing and Forecasting System, SOCIB. https://doi.org/10.25704/17GS-2B59, 2020.
Trevisanut, S.: Search and Rescue Operations in the Mediterranean: Factor of Cooperation or Conflict?, Int. J. Mar. Coast. Law, 25, 523–542, https://doi.org/10.1163/157180810X526754, 2010.
Tudor, M., Ivatek-Šahdan, S., Stanešić, A., Horvath, K., Bajić, A.: Forecasting weather in Croatia using ALADIN numerical weather prediction model, in: Climate Change and Regional/Local Responses, edited by: Ray, P. and Zhang, Y., InTech, Rijeka, Croatia, 59–88, 2013.
Ullman, D. S., O'Donnell, J., Kohut, J., Fake, T., and Allen, A.: Trajectory prediction using HF radar surface currents: Monte Carlo simulations of prediction uncertainties, J. Geophys. Res.-Oceans, 111, 1–14, https://doi.org/10.1029/2006JC003715, 2006.
Uttieri, M., Cianelli, D., Nardelli, B. B., Buonocore, B., Falco, P., Colella, S., and Zambianchi, E.: Multiplatform observation of the surface circulation in the Gulf of Naples (Southern Tyrrhenian Sea), Ocean Dynam., 61, 779–796, https://doi.org/10.1007/s10236-011-0401-z, 2011.
Vandenbulcke, L., Beckers, J. M., and Barth, A.: Correction of inertial oscillations by assimilation of HF radar data in a model of the Ligurian Sea, Ocean Dynam., 67, 117–135, https://doi.org/10.1007/s10236-016-1012-5, 2017.
Vignudelli, S., Birol, F., Benveniste, J., Fu, L.L., Picot, N., Raynal, M., and Roinard, H.: Satellite Altimetry Measurements of Sea Level in the Coastal Zone, Surv. Geophys., 40, 1319–1349, https://doi.org/10.1007/s10712-019-09569-1, 2019.
Vignudelli, S., Cipollini, P., Astraldi, M., Gasparini, G. P., and Manzella, G.: Integrated use of altimeter and in situ data for understanding the water exchanges between the Tyrrhenian and Ligurian Seas, J. Geophys. Res., 105, 19633–19649, 2000.
Vilibić, I. and Šepić, J.: Destructive meteotsunamis along the eastern Adriatic coast: Overview, Phys. Chem. Earth 34, 904–917,https://doi.org/10.1016/j.pce.2009.08.004, 2009.
Vilibić, I., Šepić, J., Mihanović, H., Kalinić, H., Cosoli, S., Janeković, I., Žagar, N., Jesenko, B., Tudor, M., Dadić, V., and Ivanković, D.: Self-Organizing Maps-based ocean currents forecasting system, Sci. Rep., 6, 22924, https://doi.org/10.1038/srep22924, 2016.
Vilibić, I., Denamiel, C., Zemunik, P., and Monserrat, S.: The Mediterranean and Black Sea meteotsunamis: an overview, Nat. Hazards, 106, 1223–1267, https://doi.org/10.1007/s11069-020-04306-, 2021.
Vučetić, T., Vilibić, I., Tinti, S., and Maramai, A.: The Great Adriatic flood of 21 June 1978 revisited: An overview of the reports, Phys. Chem. Earth, 34, 894–903, https://doi.org/10.1016/j.pce.2009.08.005, 2009.
Wang, X. and Liu, P. L.-F.: A Numerical Investigation of Boumerdes-Zemmouri (Algeria) Earthquake and Tsunami, Comput. Model. Eng. Sci., 10, 171–184, https://doi.org/10.3970/cmes.2005.010.171, 2005.
Weiss, J.: The dynamics of enstrophy transfer in two-dimensional hydrodynamics, Phys. D Nonlinear Phenom., 48, 273–294, https://doi.org/10.1016/0167-2789(91)90088-Q, 1991.
Wilkin, J. L. and Hunter, E. J.: An assessment of the skill of real-time models of Mid-Atlantic Bight continental shelf circulation, J. Geophys. Res.-Oceans, 118, 2919–2933, https://doi.org/10.1002/jgrc.20223, 2013.
Wyatt, L. R.: Wave mapping with HF radar, in: 2011 IEEE/OES 10th Curr. Waves Turbul. Meas., 25–30, 2011.
Wyatt, L. R.: Use of HF radar for marine renewable applications, in 2012 Oceans – Yeosu, 1–5, https://doi.org/10.1109/OCEANS-Yeosu.2012.6263439, 2012.
Wyatt, L. R.: High frequency radar applications in coastal monitoring, planning and engineering, Aust. J. Civ. Eng., 12, 1–15, https://doi.org/10.7158/14488353.2014.11463992, 2014.
Wyatt, L.: Spatio-Temporal Metocean Measurements for Offshore Wind Power, J. Energy Power Technol., 3, 15, https://doi.org/10.21926/jept.2101005, 2021.
Wyatt, L. R. and Green, J. J.: Measuring high and low waves with HF radar, Ocean, 2009-EUROPE, 1–5, https://eprints.whiterose.ac.uk/10590/ (last access: 9 May 2022), 2009.
Wyatt, L. R., Green, J. J., Middleditch, A., Moorhead, M. D., Howarth, J., Holt, M., and Keogh, S.: Operational Wave, Current, and Wind Measurements With the Pisces HF Radar, IEEE J. Ocean. Eng., 31, 819–834, https://doi.org/10.1109/JOE.2006.888378, 2006.
Yaremchuk, M. and Sentchev, A.: A combined EOF/variational approach for mapping radar-derived sea surface currents, Cont. Shelf Res., 31, 758–768, https://doi.org/10.1016/j.csr.2011.01.009, 2011.
Zelenke, B.: An Empirical Statistical Model Relating Winds and Ocean Surface Currents: Implications for Short-term Current Forecasts, MSc Thesis, Oregon State University, https://ir.library.oregonstate.edu/concern/graduate_thesis_or_dissertations/ms35tb992 (last access: 9 May 2022), 2005.
Zemunik, P., Bonanno, A., Mazzola, S., Giacalone, G., Fontana, I., Genovese, S., Basilone, G., Candela, J., Šepić, J., Vilibić, I., and Aronica, S.: Observing meteotsunamis (“Marrobbio”) on the southwestern coast of Sicily, Nat. Hazards, 106, 1337–1363, https://doi.org/10.1007/s11069-020-04303-2, 2020.
Zeng, Y., Zhou, H., Roarty, H., and Wen, B.: Wind Speed Inversion in High Frequency Radar Based on Neural Network, edited by: El-Darymli, K., Int. J. Antennas Propag., 2016, 2706521, https://doi.org/10.1155/2016/2706521, 2016.
Zeng, Y., Zhou, H., Lai, Y., and Wen, B.: Wind-Direction Mapping With a Modified Wind Spreading Function by Broad-Beam High-Frequency Radar, IEEE Geosci. Remote Sens. Lett., 15, 679–683, 2018.
- Abstract
- Coastal monitoring to support blue growth in the Mediterranean Sea
- High-frequency radar applications in the Mediterranean
- Discussion and preliminary assessment of the HFR regional capabilities
- Future prospects for HFR applications and recommendations
- Summary and conclusions
- Code availability
- Data availability
- Author contributions
- Competing interests
- Disclaimer
- Special issue statement
- Acknowledgements
- Financial support
- Review statement
- References
- Supplement
- Abstract
- Coastal monitoring to support blue growth in the Mediterranean Sea
- High-frequency radar applications in the Mediterranean
- Discussion and preliminary assessment of the HFR regional capabilities
- Future prospects for HFR applications and recommendations
- Summary and conclusions
- Code availability
- Data availability
- Author contributions
- Competing interests
- Disclaimer
- Special issue statement
- Acknowledgements
- Financial support
- Review statement
- References
- Supplement