the Creative Commons Attribution 4.0 License.
the Creative Commons Attribution 4.0 License.
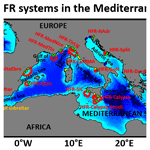
Coastal high-frequency radars in the Mediterranean – Part 1: Status of operations and a framework for future development
Pablo Lorente
Eva Aguiar
Michele Bendoni
Maristella Berta
Carlo Brandini
Alejandro Cáceres-Euse
Fulvio Capodici
Daniela Cianelli
Giuseppe Ciraolo
Lorenzo Corgnati
Vlado Dadić
Bartolomeo Doronzo
Aldo Drago
Dylan Dumas
Pierpaolo Falco
Maria Fattorini
Adam Gauci
Roberto Gómez
Annalisa Griffa
Charles-Antoine Guérin
Ismael Hernández-Carrasco
Jaime Hernández-Lasheras
Matjaž Ličer
Marcello G. Magaldi
Carlo Mantovani
Hrvoje Mihanović
Anne Molcard
Baptiste Mourre
Alejandro Orfila
Adèle Révelard
Emma Reyes
Jorge Sánchez
Simona Saviano
Roberta Sciascia
Stefano Taddei
Joaquín Tintoré
Yaron Toledo
Laura Ursella
Marco Uttieri
Ivica Vilibić
Enrico Zambianchi
Vanessa Cardin
Due to the semi-enclosed nature of the Mediterranean Sea, natural disasters and anthropogenic activities impose stronger pressures on its coastal ecosystems than in any other sea of the world. With the aim of responding adequately to science priorities and societal challenges, littoral waters must be effectively monitored with high-frequency radar (HFR) systems. This land-based remote sensing technology can provide, in near-real time, fine-resolution maps of the surface circulation over broad coastal areas, along with reliable directional wave and wind information. The main goal of this work is to showcase the current status of the Mediterranean HFR network and the future roadmap for orchestrated actions. Ongoing collaborative efforts and recent progress of this regional alliance are not only described but also connected with other European initiatives and global frameworks, highlighting the advantages of this cost-effective instrument for the multi-parameter monitoring of the sea state. Coordinated endeavors between HFR operators from different multi-disciplinary institutions are mandatory to reach a mature stage at both national and regional levels, striving to do the following: (i) harmonize deployment and maintenance practices; (ii) standardize data, metadata, and quality control procedures; (iii) centralize data management, visualization, and access platforms; and (iv) develop practical applications of societal benefit that can be used for strategic planning and informed decision-making in the Mediterranean marine environment. Such fit-for-purpose applications can serve for search and rescue operations, safe vessel navigation, tracking of marine pollutants, the monitoring of extreme events, the investigation of transport processes, and the connectivity between offshore waters and coastal ecosystems. Finally, future prospects within the Mediterranean framework are discussed along with a wealth of socioeconomic, technical, and scientific challenges to be faced during the implementation of this integrated HFR regional network.
- Article
(2058 KB) - Full-text XML
- Companion paper
- BibTeX
- EndNote
1.1 The oceanographic landscape
The Mediterranean Sea is located at the crossroads of three continents (Africa, Europe, and Asia), thereby playing an important geopolitical role in the world chessboard since ancient times as a busy navigable route for maritime transport, commerce, and cultural exchange (Gaiser and Hribar, 2012). It is a semi-enclosed, microtidal basin connected to the Atlantic Ocean, the Black Sea, and the Red Sea by three geostrategic choke points: the Strait of Gibraltar (in the west), the Dardanelles (in the northeast), and the Suez Canal (in the southeast), respectively (Fig. 1). It is also an oligotrophic well-oxygenated system characterized by complex physical and biological dynamics (Christaki et al., 2011). Offshore waters exhibit extremely low biological productivity, with the concentration of nutrients decreasing from NW to SE. The panoramic picture of the Mediterranean circulation, which exhibits a strong seasonal and interannual variability, is composed of a variety of relevant processes interacting at diverse timescales, namely water mass formation, overturning circulation, boundary currents, and frontal instabilities (Pinardi et al., 2019; Tintoré et al., 2019). The large-scale thermohaline circulation is interconnected with recurrent sub-basin gyres and energetic mesoscale eddies, which are in turn bounded by current meanders and bifurcating jets (Millot and Taupier-Letage, 2005). The rugged configuration of narrow shelf areas, with steep continental breaks, entails the intrusion and direct impact of the large-scale open-ocean flow on the coastal dynamics. For further details about general oceanographic conditions in the entire basin, the reader is referred to Pinardi et al. (2006) and Malanotte-Rizzoli et al. (2014).
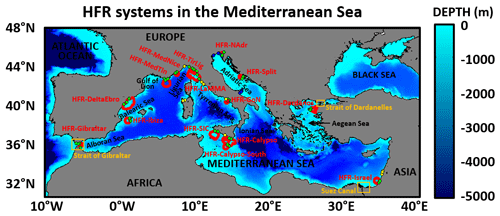
Figure 1Bathymetric map of the Mediterranean Sea, depicting some local seas and geographical features. The location and spatial coverage of ongoing high-frequency radar (HFR) systems deployed in the basin are represented with red contours. Ongoing, old, and future HFR sites are represented with green, blue, and yellow dots, respectively.
1.2 Science priorities
The Mediterranean Sea is one of the biggest reservoirs of marine life in the world, contributing to more than 7 % of world's marine biodiversity including a high percentage of endemic species (Coll et al., 2010), while only covering 0.7 % of the ocean's surface area. Since natural disasters, anthropogenic activities, and climate change might impose significant and long-lasting pressures (Juza and Tintoré, 2021; Tuel and Eltahir, 2020; Spalding et al., 2014), diverse science priorities have been identified to promote healthy and sustainable marine ecosystems in the Mediterranean Sea, including the following three among others.
The first is the detailed investigation of transport processes and the connectivity between offshore waters and coastal ecosystems. The cross-shelf exchange of nutrients, organic matter, pollutants, and other passive tracers might have relevant implications in terms of intense biogeochemical activity, eutrophication, proliferation of harmful algal blooms, and fisheries production. Equally, a deeper understanding of the ocean circulation can lead to more accurate model predictions of Lagrangian trajectories, which in turn can be used to gain insight into particle tracking, dispersion processes, residence times, and water renewal mechanisms.
The second is the impact assessment of coastal hazards and extreme sea states, ranging from storm surges, erosion, and flash floods to rogue waves and Mediterranean hurricanes, also named “Medicanes” (Von Schuckmann et al., 2020; Milglietta and Rotunno, 2019; Wolff et al., 2018; Cavaleri et al., 2012). In this context, impact assessment should be understood as the analysis of the primary met-ocean factors that give rise to severe coastal disasters and the comprehensive evaluation of the environmental effects on marine resources along with other inherent societal and economic consequences with the final aim of implementing strategic preparedness policies that could reduce both exposure and coastal vulnerability.
The third is a thorough analysis of climate-driven variations such as sea level rise, steady acidification, the increase in ocean heat content, recurrent marine heat waves, or potential alterations in the thermohaline circulation (Juza and Tintoré, 2021; Garrabou et al., 2019).
1.3 Societal challenges
The aforementioned science priorities are particularly motivated by the semi-enclosed nature of the Mediterranean Sea, where anthropogenic pressures are likely more intense than in any other sea of the world (Lejeusne et al., 2010). An increasingly high density of inhabitants (above 470 million) gravitate toward littoral regions for their living needs (Wolff et al., 2020), which are not only impacted by local human activities but also further altered by massive international tourism, including passenger ferries, cruises, and recreational boating. Apart from the shortage of water resources (tied to the population growth and the intensification of coastal urbanization, agricultural development, and industrial activities), other interconnected societal challenges in the Mediterranean Sea have been documented (Tintoré et al., 2019; López-Jurado et al., 2015) and include the following.
Enhanced maritime safety. Efficient ship routing is required to minimize both fuel consumption and the risk of accidental oil spills. Furthermore, search and rescue (SAR) operations constitute a major humanitarian emergency in the Mediterranean basin and thereby demand science-based management protocols for a timely response.
Improved ecological decision support systems. The preservation of local marine fauna (seriously jeopardized by intense overfishing), habitat modification, the transfer of alien species, and the ingestion of litter demand tailored tools for informed decision-making (Campanale et al., 2019). Equally, the monitoring of water quality in the Mediterranean Sea remains a priority, since it is negatively impacted by the discharge of land-based toxic pollutants from local rivers into coastal sea waters (Nikolaidis et al., 2014) and also by episodic marine pollution episodes (Soussi et al., 2020).
1.4 Multi-platform observing systems: high-frequency radar as a key component
To adequately respond to those science priorities and societal challenges previously enumerated, a sustainable multi-platform observing infrastructure should be implemented and integrated. The accurate monitoring and deep understanding of the Mediterranean marine environment are not only crucial to prompt a wealth of anticipatory adaptation strategies but also of great economic value for the maritime sector (Melet et al., 2020). Such preventive approaches can help to bridge the gap between marine citizen science and coastal management (Turicchia et al., 2021), which would strengthen community resilience at multiple scales (Summers et al., 2018; Linnenluecke et al., 2012).
With the advent of new technologies and ships supporting interdisciplinary suites of sensors (Mahadevan et al., 2020), a growing wealth of observational data are nowadays available to properly characterize the Mediterranean Sea (Le Traon et al., 2013). Most of these data are regularly ingested by the Copernicus Marine Environment Monitoring Service In Situ Thematic Center, hereinafter CMEMS-INSTAC (Le Traon et al., 2017), the EMODnet program (Martín Miguez et al., 2019), and the SeaDataCloud (Fichaut and Schaap, 2016), promoting an ocean observing value chain that links observations and data discovery to downstream applications for societal benefit.
For instance, two programs that contribute to the wealth of data collected in the Mediterranean Sea include novel satellite missions such as the Soil Moisture and Ocean Salinity (SMOS) and the Surface Water and Ocean Topography (SWOT), which aim to increase the resolution capacity in the coastal band to respectively properly feature the salinity field (Olmedo et al., 2018) and the submesoscale circulation (Gómez-Navarro et al., 2018). Arrays of Argo profiling floats, which provide temperature and salinity measurements down to 2000 m (Kassis and Korres, 2020; Sánchez-Román et al., 2017), are nowadays extended to the deep ocean and further complemented with data from biogeochemical Argo (D'Ortenzio et al., 2020) and biophysical gliders (Cotroneo et al., 2019; Barceló-Llull et al., 2019).
In situ measurements provided by conventional instruments such as pointwise current meters (PCMs), acoustic Doppler current profilers (ADCPs), or drifting buoys (Sotillo et al., 2016) are useful to monitor the Mediterranean circulation, but present some limitations in terms of spatial resolution as well as areal and endurance coverage. A complementary and relatively novel technology that has been steadily gaining worldwide recognition as an effective shore-based remote sensing instrument is high-frequency radar (HFR). HFR networks have become an essential component of coastal ocean observation since they collect, in near-real time, fine-resolution maps of the surface circulation over broad coastal areas, thereby providing a dynamical framework for other traditional in situ observation platforms (Roarty et al., 2019; Rubio et al., 2017). They provide two-dimensional synoptic maps of surface currents for distances up to 200 km offshore over a wide variety of high spatial (0.2–6 km) and temporal (usually between 15 min and 1 h averages) scales, enabling the detailed monitoring of (sub)mesoscale coastal processes. Although HFR-derived wave and wind measurements are not yet seen as operational products, there are many publications that demonstrate these capabilities (Esposito et al., 2018; Wyatt, 2006, 2018).
Additionally, HFR data present a broad range of science-based applications of societal benefit, such as maritime security (safe vessel navigation and SAR operations), tracking the dispersion and retention of marine pollutants (oil spill mitigation), effective monitoring of extreme events, and fisheries and coastal management (e.g., port activity and impact on marine protected areas). Other emerging uses include vessel tracking, ocean energy production, or even tsunami detection (Roarty et al., 2013; Lipa et al., 2012).
Finally, it is worth mentioning that a combined use of multi-platform observing systems, encompassing both in situ (buoys, ADCPs, drifters, tide gauges, etc.) and remote (HFRs, altimetry products, etc.) sensors, can provide additional insight into the comprehensive three-dimensional characterization of the Mediterranean Sea state at multiple scales. Equally, it can also contribute positively to a more exhaustive skill assessment of hydrodynamic, biogeochemical, and wave forecast systems running operationally in this regional basin (Aguiar et al., 2020; Mourre et al., 2018; Lorente et al., 2016a, b, 2019). The implementation of consistent data assimilation schemes has constituted a quantum leap in terms of realistic forecast predictions in the Mediterranean Sea since they maximize the interconnection of ocean observing systems and numerical models (Teruzzi et al., 2018; Dobricic and Pinardi, 2008). In this context, the benefits of assimilating HFR current data to improve ocean model forecasts in the Mediterranean region have also been demonstrated (Hernández-Lasheras et al., 2021; Vandenbulcke et al., 2017; Marmain et al., 2014).
1.5 The Mediterranean oceanography network
The Mediterranean Oceanography Network (http://www.mongoos.eu/, last access: 31 March 2022), together with EuroGOOS, is part of the 13 Global Regional Alliances of the Global Ocean Observing System (GOOS) that aims to develop both sustained ocean monitoring and tailored services to meet regional and national priorities, aligning the global goals of GOOS (https://www.goosocean.org/, last access: 31 March 2022) with the implementation of fit-for-purpose applications to satisfy local requirements (Moltmann et al., 2019). At the European level, MONGOOS plays a key role as one of the five Regional Operational Oceanographic Systems (ROOS) of EuroGOOS, helping to bridge the gap between the northern (Europe) and southern (Africa) shores of the Mediterranean Sea.
It was established in 2012 as a collaborative framework to further develop operational oceanography and sustained observations collection in the Mediterranean Sea. The network, based on its scientific and strategic plan (Sarantis et al., 2018), boosts a science-oriented vision as well as the technological developments necessary to efficiently promote regional monitoring capabilities in the Mediterranean area.
MONGOOS engages in activities related to scientific promotion, the fostering of applications for societal benefits, and the production and use of operational oceanography services. Its science and strategy plan is fully aligned with the BlueMED implementation plan (Fig. 2), within which the establishment of a fully integrated multi-platform monitoring system was acknowledged as crucial to develop a sustainable blue economy in the Mediterranean area (Trincardi et al., 2020). Furthermore, it is also in line with the EU-2020 Green Deal call named the “Digital Twin of the Ocean”. It consists of the integration of existing leading-edge capacities in ocean observation and forecasting with top-tier digital technologies (cloud infrastructures, supercomputing resources, artificial intelligence, etc.) to adequately provide a high-resolution, three-dimensional description of the ocean state in near-real time.
MONGOOS also contributes to the Decade of Ocean Science for sustainable development (2021–2030) initiative, which was proclaimed by the United Nations and relies on sustained ocean observations. It aims to create partnerships, strengthen international cooperation, mobilize resources, engage governments (and targeted stakeholders), and support high-stakes decision-making in the marine environment (Ryabinin et al., 2019). The network plays an important role in “The Science We Need for the Mediterranean Sea We Want” program (SciNMeet) recently endorsed in the first call for decade action and which encompasses a broad scope and high ambition to tackle all major environmental and social challenges in the Mediterranean basin (Fig. 2).
The MONGOOS network is formed by three working groups in charge of fostering the activity in specific areas, namely the observation, modeling, and application working groups. The Mediterranean HFR network, with participation by seven countries (Israel, Croatia, Slovenia, Malta, Italy, France, and Spain), has become an essential component of the Mediterranean oceanography network. These infrastructures are key elements for coastal observing systems providing near-real-time ocean currents with direct implications for monitoring large (regional) areas. Present applications include (i) maritime safety, (ii) extreme hazards, and (iii) environmental transport processes, which will be reviewed in a companion paper.
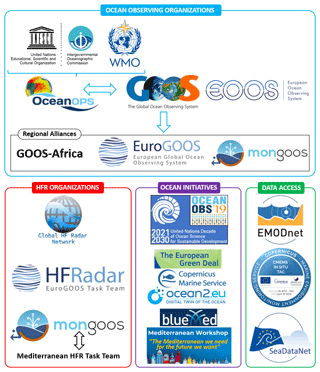
Figure 2Conceptual framework for ocean observing systems, alliances, and initiatives ranging from global to regional scales. OceanOPS, which depends on both the UNESCO Intergovernmental Oceanographic Commission and the World Meteorological Organization, represents the operational center of GOOS where meteo-oceanographic observing systems are centralized. The double-sided arrow between MONGOOS and the Mediterranean HFR task team intends to highlight the two-way interaction between the two entities: the former sets specific tasks and the general strategy, while the latter provides data and support to update the predefined roadmap.
1.6 Objectives of the work
Motivated by the increasing relevance of the consolidated HFR technology, this work pursues several interrelated goals.
- i.
Showcase the current status of the Mediterranean HFR network, providing a succinct description of each HFR system. Ongoing work plans, recent progress in basic products, and applications are enumerated, thereby highlighting the benefits of this cost-effective technology for the multi-parameter monitoring of coastal waters.
- ii.
Show the links of this HFR network with diverse multi-institutional initiatives and alliances at regional and global level, emphasizing the bidirectional interactions with the global HFR network (Roarty et al., 2019), the EuroGOOS HFR task team (Rubio et al., 2017), GOOS, and EuroGOOS (Fig. 2). Equally, the connections with other European initiatives such as the Copernicus Marine Environment Monitoring Service – CMEMS (Le Traon et al., 2017) and cross-border projects (e.g., EuroSea, JERICO-NEXT, IMPACT, SICOMAR plus, SINAPSI, CALYPSO) are also presented.
- iii.
Delineate future prospects within the Mediterranean framework along with the various challenges to be faced, encompassing economic, technical, and scientific aspects. In this context, the Mediterranean framework should be understood as the ocean observational infrastructure already implemented together with a range of thematic areas (gaps, resources, dilemmas, strategic issues, etc.) that inherently shape coordinated efforts and the future roadmap of the MONGOOS HFR team.
This paper, which constitutes the first part of a double contribution, aims to provide a panoramic overview of the roadmap to transform individual HFR systems into a fully integrated, mature network operated permanently in the Mediterranean Sea. The second part focuses on the latest scientific breakthroughs and diverse research-based applications of HFR data, fully aligned with predefined science priorities, in order to meet both societal needs and stakeholder demands in an innovative way (Reyes et al., 2022).
The paper is organized as follows. Section 2 describes the fundamentals of HFR technology and basic HFR-derived products, encompassing the retrieval of surface currents, wave parameters, and directional wind estimations. Sections 3 and 4 outline fundamental technical aspects of each HFR system within this regional network and a number of collaborative projects, respectively. Ongoing and future challenges to be faced over the next decade are discussed in Sect. 5. Finally, main conclusions are drawn in Sect. 6.
2.1 Fundamentals of HFR technology
HFR technology, founded on the principle of Bragg scattering of the electromagnetic radiation over the rough conductive sea surface (Crombie, 1955), infers the radial current component from the Doppler shift of radio waves backscattered by surface gravity waves of half their electromagnetic wavelength. Each single radar site is configured to estimate radial currents moving toward or away from the receive antenna. Since the speed of the wave is easily derived from linear wave theory, the velocity of the underlying ocean surface currents can be computed by subtraction. The distance to the backscattered signal is determined by range-gating the returns. Although all HFR systems rely on fundamentally similar physics and Doppler processing algorithms to infer the range and radial velocity of the scattering surface, they differ in the reception and interpretation of the incoming direction of the backscattered signal.
According to the methodology used to determine the incoming direction of the scattered signal (also named “bearing determination”), commercial HFR systems can be differentiated into two major types: beam forming (BF) and direction finding (DF). BF radars use linear phased arrays of receive antennas (typically between 8 and 16 antennas in a linear array) to electronically point towards a sector of ocean surface, which amplifies signal strength from that direction and attenuates the signal from other directions. The WEllen RAdar (WERA), developed by the University of Hamburg and manufactured by Helzel Messtechnik GmbH (Gurgel et al., 1999), is one example of a BF radar. DF radars, such as the Coastal Ocean Dynamics Application Radar (CODAR) SeaSonde (Barrick et al., 1985), measure the return signal continuously over all angles, exploiting the directional properties of a three-element antenna system (two directionally dependent orthogonal crossed loops and a single omnidirectional monopole) and use the Multiple Signal Characterization (MUSIC) DF algorithm (Schmidt, 1986) in order to determine the direction of the incoming signals.
A large number of HFR systems are active worldwide operating at specific frequencies within the 3–30 MHz band and providing radial measurements which are representative of current velocities in the upper 0.5–4 m of the water column (further details can be found in Rubio et al., 2017). In regions of overlapping coverage from two or more sites, radial current estimations are geometrically combined to estimate total current vectors on a predefined Cartesian regular grid. The specific geometry of the HFR domain and, hence, the intersection angles of radial vectors influence the accuracy of the total current vectors resolved at each grid point. Such a source of uncertainty is quantified by a dimensionless parameter denominated as the geometrical dilution of precision or GDOP (Chapman and Graber, 1997), which typically increases with distance from the HFR sites.
Another relevant difference among HFR systems is the way the signal is transmitted and received. Typically, HFRs transmit a using frequency-modulated continuous wave (FMCW), which consists of a signal whose frequency is linearly swept (also called chirp signal). Using pure FMCW, the transmitter and receiver antennas are constantly transmitting and receiving. This means that the receiver antenna has to be physically separated from the transmitter to reduce direct leak of the transmitted signal into the receivers, which may saturate the electronics. Compact versions of HFR implement interrupted FMCW (iFMCW or FMiCW) in which the transmit signal is switched off and on repetitively. Under this scheme, the receivers process backscattered information from the off-state of transmission only. This improves the isolation of direct leakage of transmitted signal into the receivers, enabling compact antenna configurations wherein the transmit and receive antennas are collocated with usage of the same antenna as both a transmitter and receiver. Some phased-array versions of HFR also implement FMiCW to avoid saturation of the receiver's analog-to-digital converter (ADC) from the strong transmitted signal, which could deteriorate the correct measurement of the signals coming from the ocean if the adequate separation between the transmitter and receiver is not taken into account.
Due to the lack of interruption on the receiver, pure FMCW harnesses more backscatter energy from the ocean, improving the range performance of the HFRs (Heron et al., 2015). Also, the type of processing impacts the integration time, which is usually higher with DF than BF. The reason is that DF processing requires a sufficient number of sample Doppler spectra (hence a longer integration time) to estimate the covariance matrix, which is at the heart of the method. Less integration time can be advantageous for extremely dynamic seas or for specific applications such as tsunami detection and ship tracking. However, for both FMCW and FMiCW (either BF or DF), reducing the integration time results in less accurate surface current outputs as averaging measurements at different levels might get rid of (i) chaotic changes due to turbulence, (ii) subgrid-scale variability of the surface current, and (iii) random fluctuations of the sea echo itself due to the Gaussian nature of the Bragg ocean waves and the linear transformation represented by the scattering from them (Barrick, 1980; Wang et al., 2014).
Phased-array systems can also employ DF techniques. The WERA system is also available in a configuration using a squared receive array of four antennas (not collocated) which employs DF techniques, although this option is not widely used. The only example in the Mediterranean Sea with such a configuration is given in Zervakis (2017). DF techniques have also been applied to linear arrays, further improving the azimuth resolution (Barbin et al., 2009; Barbin, 2011). More recently, some operational applications have been developed in the Mediterranean Sea by using a hybrid approach that applies both BF (antenna grouping) and DF techniques to phased-array HFR systems (Dumas et al., 2020).
Robust surface current measurements can be derived from the Doppler shift of the dominant first-order peak in the radar echo spectrum (Stewart and Joy, 1974). The use of first-order peaks to measure wind direction, albeit less explored, has been previously reported in the literature (Heron, 2002; Lipa et al., 2014; Kirincich, 2016; Hisaki, 2017; Shen and Gurgel, 2018; Wyatt, 2018; Saviano, 2021). The directional wave spectrum and derived parameters such as local significant wave height, centroid wave period and mean wave direction can be determined from the weaker second-order sea-echo Doppler spectrum by adopting two main approaches: full integral inversion or fitting with a model of ocean wave spectrum (Lipa and Nyden, 2005). A variety of inverse techniques have been developed over the last years (Barrick, 1977; Wyatt, 1990; Hisaki, 2006).
The second-order scattering-based methods significantly rely on the echo quality, which varies with sea state and radar frequency (Wyatt et al., 2005). The relative contribution of the second-order spectrum increases with both the radar frequency and the wave height. Since wave data are dependent upon the occurrence of both Bragg and larger surface gravity waves, there is a minimum threshold for sea states at a given radar frequency in which reliable wave parameters can be determined. Below such a sensitivity threshold, the lower-energy second-order spectrum is closer to the noise floor and more likely to be contaminated with spurious contributions that might result in wave height overestimation or limited temporal continuity in wave measurements (Lipa and Nyden, 2005; Tian et al., 2017). During extreme weather events, there is also a limiting factor for HFR accuracy as the wave height increases and exceeds the saturation limit defined (on an inverse proportion) by the radar transmit frequency. If the radar spectrum saturates, the first-order peak merges with the second-order one and interpretation of the spectra becomes impossible with existing methods (Forney et al., 2015).
The development of robust validation methodologies constitutes a core activity when implementing a fully operational network since HFR measurements might be subject to some error sources and potential uncertainties. Inherent problems of HFR technology, such as power-line disturbances, radio frequency interference, ionospheric clutter, environmental noise, unresolved velocity fluctuations, reflections from moving ships, offshore wind turbine interference, adverse environmental conditions, improper determination of the angle of arrival, limitations in signal processing methods, antenna pattern distortions, or hardware failures, likely impact the accuracy of HFR measurements (Paduan et al., 2006; Kohut and Glenn, 2003). Since HFR is gaining ever-wider acceptance by the oceanographic community as an efficient land-based technology for the multi-parameter monitoring of the sea state in near-real time, it is essential to routinely assess the accuracy of HFR measurements against independent in situ observations, fostering subsequent use for research purposes and the development of added-value operational tools.
2.2 Basic HFR products
2.2.1 High-Frequency radar surface current monitoring, improvement, and validation
The primary goal of oceanographic HFR systems is the derivation of radial and total ocean surface currents from the backscattered signal on the receiving antennas. The measurement principle relies on the first-order “Bragg theory” according to which the dominant contribution to the backscattered electromagnetic field is the resonant surface wave with half-radar wavelength. This results in a couple of sharp peaks in the positive and negative part of the Doppler spectrum located at the so-called “Bragg frequency” and its opposite. This remarkable property was first experimentally observed by Crombie (1955) and given a solid theoretical framework by Barrick (1972). It was later realized that this could be used to infer the radial surface current by measuring the frequency shift between the theoretical and observed positions of the Bragg peaks (Stewart and Joy, 1974).
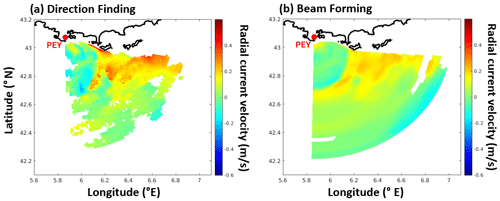
Figure 3Hourly radial surface current maps obtained on 1 September 2020 at 06:00 TU with the HFR station deployed in Fort Peyras (PEY) in Toulon (SE France) with a 12-antenna receiving array operating at 16.15 MHz. The range resolution is 1.5 km and the maximum range is about 80 km. (a) Radial map obtained by using antenna grouping and self-calibration: fine and contrasted structures are unveiled. (b) Radial map obtained using the classical beam forming azimuthal processing: small patterns are smoothed and contrast is reduced.
Despite the simplicity of the physical concept, the estimation of the radial current in every sea surface patch from the mere antenna voltage requires a chain of technical and processing steps which are far from being trivial. It also implies choices and compromises from the operator depending on the logistical constraints and the planned applications. The main limitation is the ability to properly identify the first-order Bragg peaks and their exact locations. This is related to the signal-to-noise ratio (SNR), the integration time, and the number of sample spectra which are combined over the observation time. The SNR is primarily dependent on the transmitted power and determines the effective range of the HFR. Increasing the integration time improves both the SNR and the Doppler resolution but reduces the number of available samples. This cannot be compensated for by an augmentation of the observation time, which is limited by the assumption of stationary currents. Another challenge is the production of surface current maps obtained by resolving the received signals in range and azimuth. While range gating is always achieved with a standard FMCW chirping technique, the azimuthal discrimination of surface currents is a more delicate task. Extended linear antenna arrays (classically done with BF techniques) allow sweeping the different bearings in the radar field of view. With cross-loop compact antenna systems, the directions of arrival are obtained through high-resolution DF methods such as the MUSIC algorithm. These are based on a covariance analysis of the individual Doppler spectra received on each antenna, an operation which requires processing a sufficient number of sample spectra (Emery, 2020). For this reason, compact systems usually necessitate a longer observation time than phased-array systems to obtain reliable surface current maps. The latter have a more irregular aspect than those obtained with BF and do not suffer from angular smoothing. However, some wrong or missing allocations of the directions of arrival can make them lacunary and spotted with many outliers. The quality of azimuthal processing with compact systems also relies on the calibration of the complex antenna gains, a procedure that usually necessitates extra hardware deployment. A last factor that impacts data quality is the frequent occurrence of radio frequency interference (RFI) from external electromagnetic sources. The RFI produces sharp artificial Doppler peaks in the direction of the source, which can be erroneously interpreted as Bragg peaks and lead to a strip of false values in the radial current map.
Overall, there are many factors that affect the “voltage to radial current” transformation and might degrade the quality of the resulting surface current maps. This often results in a poor spatial coverage due to lacunary-estimated and limited SNR, outliers due to wrong allocations of direction of arrivals or RFI, and smoothed, underestimated currents due to an insufficient angular (BF) or temporal (DF) resolution.
To mitigate these deficiencies the HFR currents generally undergo some a posteriori processing and quality checks as described in Mantovani et al. (2020). However, very often this cannot fully compensate for the insufficient quality and coverage of data and can even produce realistic looking but incorrect artificial maps. It is therefore important to correct as much as possible the shortcomings of HFR currents at the early stage of the voltage to current transformation in order to optimize a posteriori processing and minimize its artifacts.
In the last few years some promising ideas and techniques have been proposed to improve the quality of raw HFR signal processing. These include new calibration techniques (e.g., Flores-Vidal et al., 2013), original antenna processing methods (e.g., Dumas and Guérin, 2020; Guérin et al., 2021), use of bistatic and multi-static configurations (e.g., Dumas et al., 2020), efficient RFI rejectors (e.g., Tian et al., 2017; Gurgel et al., 2007), and non-spectral estimators (e.g., Domps et al., 2020, 2021). Figure 3 shows an example of the amelioration that can be obtained with a non-standard array processing method based on antenna grouping and direction finding (Dumas and Guérin, 2020) over a classical beam forming in the case of the 12-antenna receiving array of Fort Peyras (Toulon, southeastern France). As seen in Fig. 3, fine contrasted patterns of radial current are unveiled when resorting to such a high-resolution technique while maintaining a good spatial coverage.
The credibility of HFR-derived current data has been extensively proven in numerous coastal areas of the Mediterranean Sea by adopting Eulerian or Lagrangian approaches. Previous investigations included direct comparisons against independent in situ sensors like PCMs, moored ADCPs, drifters, ship-based sensors, or similar (Cosoli et al., 2010; Berta et al., 2014; Lorente et al., 2014, 2015, 2021; Corgnati et al., 2019a; Lana et al., 2016; Kalampokis et al., 2016; Capodici et al., 2019; Guérin et al., 2021, Molcard et al., 2009; Bellomo et al., 2015).
When the HFR footprint overlooks a moored instrument within its spatial coverage (Fig. 4a), an accuracy assessment of HFR surface currents can be performed with radial or total vectors. In the first case, the HFR radial arc geographically closest to the in situ instrument location is selected for each HFR site, and radial current vectors estimated at each arc point are compared with the radial projection of PCM velocities (Cosoli et al., 2010; Lorente et al., 2014, 2015). This comparative analysis allows the computation of statistical parameters (e.g., the correlation – CORR – and the root mean squared error – RMSE) as a function of the angle between the buoy and the arc grid point position. In the absence of direction-finding (DF) errors, maximum CORR and minimum RMSE values should be found over the arc point closest to the buoy location. In the presence of DF, the bearing offset is thus expressed as the angular difference between the arc point with maximum correlation and the buoy location (Fig. 4b). In the second case, HFR total vector hourly estimations at the grid point closest to the buoy location are compared against in situ current data to provide upper bounds on the HFR accuracy. Comparisons are commonly undertaken using zonal (U) and meridional (V) components in order to evaluate the agreement between the two instruments (Fig. 4c).
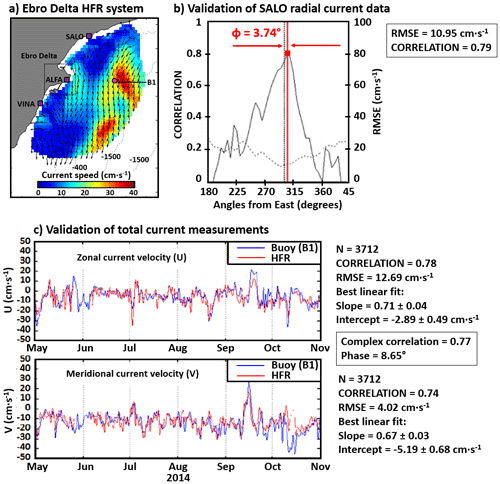
Figure 4Validation of hourly surface currents provided by the HFR deployed in Ebro Delta (NW Mediterranean Sea), shown in Fig. 1, against a buoy for May–October 2014 (Lorente et al., 2015). (a) Example of an hourly map of surface current circulation. The pink dot and purple squares represent the buoy and HFR site locations, respectively. (b) Validation of radial currents. Correlation (solid line) and RMSE (dashed line) between radial currents estimated by the buoy and those measured by the SALO HFR site. The vertical dotted line represents the angular position of the moored buoy. The vertical red solid line denotes the angular position of maximum correlation, which is gathered with the associated RMSE and bearing offset (in red) values. (c) Low-pass-filtered time series of zonal and meridional currents measured by the buoy (blue line) and HFR at the closest grid point (red line).
Supplementary validation works with radial measurements were carried out in the Mediterranean Sea when the geometry of the emplacement gave the chance to perform a self-consistency analysis of the radar-to-radar overwater baselines in order to evaluate intrinsic velocity uncertainties in HFR radial velocities (Lorente et al., 2014). This methodology, previously applied in other parts of the world (Paduan et al., 2006; Atwater and Heron, 2010; Gómez et al., 2020), states that in the absence of errors two facing HFR sites should provide the same estimates of radial velocities (differing only in the sign) at the midpoint of the baseline that joins them, since the range and the angular distribution are similar. This self-consistency test presents some benefits like the nonexistence of horizontal-scale or depth mismatch, as the two involved sites are operating in the same frequency, providing two current datasets with, in principle, identical origin and nature.
In terms of Lagrangian assessment, it is worth mentioning that the Tracking Oil Spill and Coastal Awareness (TOSCA) project experience (Bellomo et al., 2015) constituted one of the first coordinated initiatives at the Mediterranean level to test the precision of a core of 12 HFRs and identify a set of good practices for pollution mitigation. Among other valuable goals, the five-country TOSCA experiment aimed at comparing HFR-derived measurements against the trajectories provided by 20 Coastal Ocean Dynamics Experiment (CODE) drifters (Davis, 1985), which were drogued in the upper 1 m of the oceanic layer and acted as proxies for substances passively advected by currents. In all cases, the RMSE of the radial velocity difference between HFR and drifters was in the range of 5–10 cm s−1, which is in line with previous literature given the expected variability at the HFR subgrid level.
As an overall summary of the validation works, RMSE and CORR values have been typically reported to fall in the ranges 5–20 cm s−1 and 0.32–0.92, respectively (Cosoli et al., 2010; Berta et al., 2014; Lorente et al., 2014, 2015, 2021; Corgnati et al., 2019a; Lana et al., 2016; Kalampokis et al., 2016; Capodici et al., 2019; Guérin et al., 2021; Molcard et al., 2009; Bellomo et al., 2015). Relative HFR velocity errors can vary widely depending on the characteristics of the site, the radar transmission frequency, the sensor type, and location within the sampled domain, as well as the data processing scheme used (Rypina et al., 2014; Kirincich et al., 2012).
These validation studies acknowledged that observed discrepancies between HFR in situ estimations might be partially attributable to the combined contribution of several factors such as the mismatch in time sampling and averaging, distinct horizontal averaging scales, contributions from Stokes drift likely included in HFR-derived estimates, or the influence of Ekman stratification in the current profile, subsequently leading to potential velocity differences in the upper water column (Laws et al., 2003; Ohlmann et al., 2007; Chapman et al., 1997; Kohut et al., 2006). In this frame, the instrumental noise and subgrid-scale current variability have also been documented to yield noise levels of 4–6 cm s−1 (Emery et al., 2004; Ohlmann et al., 2007; de Paolo et al., 2015).
Once HFR has proven to be a valid instrument to accurately monitor surface currents with high spatiotemporal resolution over wide coastal areas, the ability of this remote sensing technology to measure waves and wind direction must also be assessed, as detailed in the next two sections.
2.2.2 Wave measurement retrieval from HFRs
In addition to surface ocean currents, HFR directional wave spectrum and derived parameters such as local significant wave height, centroid wave period, and mean wave direction can be determined from the weaker second-order sea-echo Doppler spectrum by adopting two main approaches: full integral inversion or fitting with a model of ocean wave spectrum (Lipa and Nyden, 2005). A variety of inverse techniques have been developed over the last decades (Barrick, 1977; Wyatt, 1990; Hisaki, 2006).
Wave measurements derived from HFR have a broad range of potential applications and can be used as input data for numerical model validation (Saviano et al., 2020a), assimilation into wave models (Siddons et al., 2009; Waters et al., 2013), wave energy harvesting (Ramos e al., 2009), or the analysis of extreme wave height events (Lorente et al., 2021). HFR wave data can provide assistance to maritime navigation and wise decision-making, from both commercial and recreational perspectives, by identifying severe sea states in densely operated maritime areas where fixed in situ moorings may be compromised (e.g., at the entrance of congested harbors, first-order spots in terms of activity and trade volume). Furthermore, HFRs can help detect the interaction between high incoming waves, intense river outflow currents, and wind-forced flow over the inner continental shelf, as highlighted by Lorente et al. (2021).
In order to infer how much confidence can be placed in wave parameters retrieved by HFR systems, their accuracy must be evaluated under different sea states and coastal configurations (Fig. 5). Previous validation experiments, some of them listed in Table 1, included comparisons against independent in situ observations, remotely sensed wave estimations, or numerical outcomes over a variety of regions in the Mediterranean Sea such as the Gulf of Naples (Falco et al., 2016; Saviano et al., 2019, 2020a, b, 2022), Sicily (Orasi et al., 2018), and the Ebro Delta (Lorente et al., 2021). Regardless of the manufacturer, the operational frequency, and the methodology used to determine wave parameters, the positive contribution of commercial HFR systems to characterize the main wave patterns (and the related spatiotemporal variability) has been unequivocally proven under both standard met-ocean conditions and severe sea states.
Table 1Review of the most recent studies about validation of wave parameters derived from high-frequency radar (HFR) against independent wave observations. Skill metrics obtained for the significant wave height (SWH) during the studied period included the mean squared error (MSE), the root mean square error (RMSE), the normalized RMSE (RMSEN), the Pearson's correlation coefficient (CORR), and the skill score (SS) proposed by Wilmott (1981). The metric intervals denote the range of results obtained for several sites composing each HFR system.
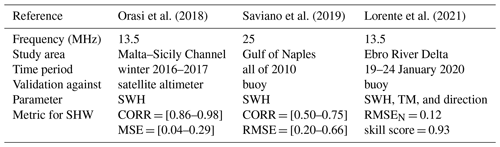
A widely accepted approach with DF systems consists of comparing HFR wave estimations, extracted along several annular rings (circular concentric range arcs) centered in each of the HFR sites, against in situ observations to quantify the degree of accuracy as a function of the distance to the shoreline. As shown by Saviano et al. (2019) and Lorente et al. (2021), wave estimations are often averaged among the intermediate range arcs to improve data quality and availability. This constitutes an optimal operational trade-off, as it guarantees the highest number of recordings. While close enough to the shoreline (so the sea-echo intensity is sufficiently high to ensure good data quality), the range arcs are also deep enough to avoid shallow-water effects on radar sea echo: wave breaking and the decrease in saturation limit on wave height as ocean depth decreases (Lipa et al., 2008).
In the case of linear phased-array BF systems consisting of at least 12 antennas, they can provide maps of wave parameters with the same spatiotemporal resolution as with surface currents (Gómez et al., 2015).
According to the skill metrics presented in Table 1, which are in accordance with previous validation exercises reported in other European waters (Basañez et al., 2019; López and Conley, 2019; Lópezet al., 2016; Gómez et al., 2015), it can be concluded that properly treated HFR-derived wave estimations can be potentially employed for operational coastal monitoring across a wide range of sea states. Ad hoc quality control methodologies, based on the particular local environment, are required to ensure robust HFR wave measurements. Although the precision and availability of HFR-derived wave estimations have been documented to be lower during calm sea states (as the second-order spectrum is closer to the noise floor), HFR might act as an effective coastal monitoring asset, especially in locations where in situ devices cannot be deployed (such as harbor entrances) or when in situ wave observations are temporarily unavailable due to instrument outages or breakdowns.
Particularly for the Mediterranean coastal waters, the performance of the HFR system installed in the Gulf of Naples (GoN hereinafter) in the retrieval of wave parameters has been tested in different works, aimed at providing, on the one hand, an assessment of the accuracy of HFR measurements and on the other a reconstruction of the wave climatology of the basin. The validation of HFR wave data has been accomplished using two alternative data sources, namely an in situ buoy and two wave models. In the first case (Saviano et al., 2019), recordings from an offshore directional buoy (located outside the areal coverage of the HFR system) were used to evaluate the agreement with the patterns depicted by the three HFR sites building the GoN network (Fig. 1) over a 1-year reference period. As reflected in Fig. 5a, the comparison indicated that both platforms returned collimating descriptions of the wave field under both calm and stormy periods and that the HFRs could also retrieve realistic measurements above the theoretical maximum recordable wave height (Lipa and Nyden, 2005). Additional insight into the validity of HFR data has been gained by the comparison against wave measurements provided by two models, WAVEWATCH III and SWAN (Simulating WAves Nearshore), over a 3-year period (Saviano et al., 2020a). Overall, the HFR and model data were consistent, although discrepancies in lower sea states and in extreme conditions could be reported. The validation of HFR measurements was a fundamental prerequisite to extract long-term information on the characteristics of the wave field in the GoN and exploit them to build a wave climatology of the basin. To this aim, wave measurements from the HFR network over 4.5 years were complemented with records from an ADCP interlocked with a Monit-Med (MEDA) elastic beacon collected over almost 3 years (Saviano et al., 2020b).
The integration of the results gathered through these works allows depicting some peculiarities of the wave field in the GoN, namely (i) a predominantly wind-driven wave field with specific seasonal recurrent patterns, (ii) the occurrence of more energetic conditions in autumn–winter, particularly in association with low-pressure systems acting over the region, (iii) the establishment of a stable calm state driven by the spring–summer breeze regime, and (iv) the directional distribution of approaching waves depending on the sub-basin of the GoN considered, corresponding to the different sectors covered by each HFR site.
These patterns are comparable over the years (Falco et al., 2016; Saviano et al., 2019, 2020a, b) but at the same time are coherent with the typical climate of the southern Tyrrhenian Sea and with previous studies carried out in the GoN. In addition to insights strictly focusing on the basin dynamics, the outcomes collected in the GoN demonstrate that the HFRs provide reliable measurements of waves, particularly in terms of significant wave height. With reference to wave period, the DF system returns a centroid period which falls between the mean and peak periods typically retrieved by other platforms (Saviano et al., 2019, 2020b). As such, the centroid period can be used as a robust estimator in line with what is discussed in Long et al. (2011). In a more general framework, the positive experience in the GoN demonstrates that HFRs should be considered an integral part of the design and implementation of coastal monitoring systems thanks to their ability to reconstruct not only the surface current field, but also wave dynamics and wind. The performance of HFR systems, however, still needs to be improved as discussed in Saviano et al. (2019), for example by standardizing QA–QC protocols and/or optimizing inversion methods and wave retrieval algorithms.
In the Malta–Sicily Channel, Orasi et al. (2018) compared significant wave height measurements from four HFR sites against both satellite altimeter data (i.e., Jason2, Jason3, and SAR SARAL/AltiKa missions) and numerical simulations. Sea state forecasts over the Mediterranean Sea were provided by the Mc-WAF system based on the WAM model with a horizontal resolution and operated by ISPRA since 2012. As shown in Fig. 5b, better agreement is achieved in the intermediate range rings, particularly when compared against altimeter data. Intermediate rings are those placed at intermediate distances from the coast, not the first one (closest to the HFR site location) nor the outermost range ring. WAM slightly underestimates the SWH during a storm event occurring along the analyzed time series with a return period of 4 years.
In terms of extreme events, record-breaking storm Gloria (19–24 January 2020) hit the NW Mediterranean Sea with heavy rainfall, strong easterly winds, and very high waves (Lorente et al., 2021). Although the low-lying Ebro Delta region (Fig. 4a) was severely inundated, the HFR deployed there was able to effectively monitor Gloria's striking features. As shown in Fig. 5c, the visual resemblance between in situ data and HFR-derived estimations of SWH (from ALFA site) is remarkably high. The peak, which was well captured in terms of intensity (7.28 m) and timing, fairly exceeded the percentile 99 derived from the buoy estimations for a 15-year period (2004–2019) established at 2.87 m.
For phased-array HFR, the reconstruction of the wave field from the backscattered signal can be attempted by using a single station (Fig. 5d). Depending on the method used, this can provide different estimations of the wave frequency spectrum, from which integrated parameters can be estimated such as significant wave height and wave period. Nevertheless, approaches inverting the nonlinear integral equation of radar cross-section and more simplified empirical approaches both result in an ambiguity of the directional spectrum solution (Hisaki, 1996; Gurgel et al., 2006). Therefore, to solve this ambiguity and to be able to provide directional wave information, a second HFR site overlooking the same ocean patch from a different direction is required.
An evaluation of wave parameters measured by a single HFR station concluded that significant wave height estimates are not robust when the waves propagate roughly perpendicular to the radar beam. In such cases, which were not presented often, a different algorithm can be used, which improves the estimations. Since there is no directional information provided by a single HFR, there is no way to select between the two algorithms solely by using the measured data. It was shown that dual-radar estimates are more accurate than using single-radar site estimations (Wyatt, 2002).
In order to solve the above discrepancy, de Valk et al. (1999) took into account additional physics. Their reconstruction method inverts the Doppler backscatter integral together with a reconstruction of the wave field using the wave action equation while neglecting ambient currents and various source functions. Hisaki (2006) extended the de Valk et al. (1999) approach to also include the wind input, dissipation, and nonlinear interaction source terms. Both require solving an iterative and location-specific model. A more recent work (Alattabi et al., 2019) provides a model which treats swell and wind waves separately, combining former works into a single empirical hybrid model. Its results using a single very high-frequency (VHF) station provided good correlation with various in situ measurements. This application has some limitations for nearshore swells, but its accuracy and simplicity show good perspectives for a large-scale adaptation after confirmations using radar systems of different frequencies.
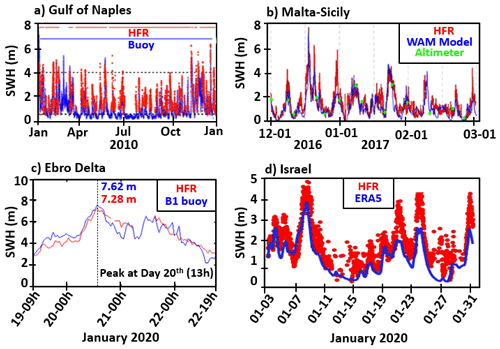
Figure 5(a) Time series of SWH provided by HFR-GoN (SORR site) and a buoy deployed in the GoN (Fig. 1). Timelines of data availability provided at the top. Dotted lines represent the theoretical upper and lower detectability thresholds of this HFR. (b) Time series of SWH (averaged in time and space) provided by HFR-Calypso (arc 3 of Barkat site), the WAM model, and an altimeter during a storm in the Malta–Sicily Channel. (c) Time series of SWH provided by HFR-DeltaEbro (arcs 4–9 of ALFA site) and the B1 buoy during storm Gloria in the NW Mediterranean Sea. (d) Time series of SWH (∼20 km offshore of Israel) as measured by HFR-Israel (Fig. 1). Preliminary uncalibrated data using Gurgel and Schlick (2006) were compared with ERA5 wave reanalysis (from the ECMWF).
A portion of the detected discrepancies in wave measurements could be attributed to the following: (i) the mathematical inversion process of the second order is unstable and diverges rapidly from the true solution in presence of noisy data; (ii) the assumptions made in the inversion method (the Pierson–Moskowitz fit-to-spectrum unimodal model used has previously proven its validity to properly describe wind-dominated seas and also swell-dominated seas, whereas this might be different under some combination of multi-modal sea states under complex met-ocean conditions); and/or (iii) the different sampling techniques. Whereas DF HFR systems provide wave data averaged over range rings (assuming homogeneity over the whole of each circular range cell), buoys give point measurements. In this context, coastal effects can also lead to locally varying wave fields and make absolute comparisons between in situ and remote sensing instruments even harder.
2.2.3 Wind measurement retrieval from HFRs
While the analysis of surface currents and the retrieval of wave parameters are well established, the worldwide use of first-order peaks to measure wind direction still remains less explored (Heron and Rose, 1986; Heron, 2002; Kirincich, 2016; Hisaki, 2017; Wyatt, 2018). Also, additional efforts should be devoted in the short-term future to the development of robust algorithms for a reliable measurement of wind speed (Shen et al., 2012; Vesecki et al., 2002).
To the best of our knowledge, Saviano et al. (2021) constitutes the first attempt to analyze HFR-derived wind direction in the Mediterranean Sea using a 25 MHz DF system. HFR measurements were compared with in situ recordings from a weather station in the GoN, revealing (i) the potentialities of this remote sensing technology as a monitoring platform when the wind speed exceeds a 5 m s−1 threshold and (ii) the relevance of the operational frequency in the accuracy of HFR wind measurements, with higher frequencies leading to estimations that are in better agreement with in situ measurements, as previously indicated by Shen and Gurgel (2018). This is due to the fact that resonant Bragg waves have a shorter wavelength and are thus more sensitive to changes in the wind direction field.
The first model to extract the wind direction from HFR backscatter was suggested by Long and Trizna (1972). In recent decades, different research groups developed algorithms for the extraction of wind direction (Zeng et al., 2018; Chu et al., 2018; Hisaki, 2017; Kirincich, 2016; Shen et al., 2012; Heron, 2002; Huang et al., 2004; Gurgel et al., 2006), and more recently a neural network method was applied for wind field inversion (Zeng et al., 2016).
Although works and publications dealing with HFR wind measurements are still scarce compared to those analyzing HFR currents or waves, several examples presenting and validating HFR wind direction data can be found in the literature (Heron, 2002; Lipa et al., 2014; Kirincich, 2016; Hisaki, 2017; Shen and Gurgel, 2018; Wyatt et al., 2001; Wyatt, 2018; Saviano, 2021).
Some of these previous studies affirm that the accuracy of HFR wind direction measurements is related to many factors (Lipa et al., 2014). Diverse studies on the comparison with in situ measurements acknowledged that with wind speeds lower than 5 m s−1 the reversal of the wind direction and hence HFR-derived wind direction is not reliable (Lipa et al., 2014; Wyatt, 2018; Shen and Gurgel, 2018). This is mainly due to the fact that at high wind speeds, the direction of the Bragg resonant waves (i.e., the HFR-derived wind direction) agrees better with the wind direction (Shen and Gurgel, 2018). Another important factor is the frequency of the HFR, since HFR systems operating at higher frequencies lead to wind direction measurements that are in better agreement with in situ ones. This is due to the fact that the corresponding resonant waves (i.e., half the radio wavelength) are relatively shorter ones, being more sensitive to a change in wind direction, rapidly responding to local wind excitation and variability (Shen and Gurgel, 2018). In addition, accurate knowledge of the seasonal wind field of the study area is fundamental to assess the correct investigation.
In the Ligurian Sea experiment, a pattern-fitting method for wind direction inversion from a 12 MHz beam forming HFR was presented in Shen and Gurgel (2018). A meteorological buoy provided the in situ wind speed data from 10 May to 8 June 2009. During the experiment, the wind speed was relatively low, and only 18.9 % of wind records exceeded 5 m s−1. Results showed that, for wind direction measurements from HFR backscatter, the accuracy strongly depends on the radar frequency, and from the measurement of wind speed using buoys, under higher wind conditions, the inversion of wind direction is better.
The analysis in the GoN (southern Tyrrhenian Sea), in an intricate coastal area with very special local factors influencing the wind field, showed comparisons between HF wind direction, in situ measurement (weather station), and the model SKIRON/Eta in selected events (Saviano et al., 2020a, b). As shown in Fig. 6, the comparisons reveal good statistical agreement between the platforms with robust values of the circular correlation coefficient during winter events when the wind speed exceeded the threshold of 5 m s−1 for a period of 72 h (for circular statistics applied to HFR data, see Ranalli et al., 2018). Furthermore, the acquisitions of all range cells (RCs) or annulus around the HFR sites were investigated: in all the events, the RCs near the coast and the offshore ones give poorer statistical results compared to the central RCs, while the best agreement is found between 4 and 10 km from the coast (Fig. 6).
Although the RMSE values obtained for wind direction in the GoN appear to be high, they are in line with similar experiments carried out previously in the Mediterranean Sea (Shen and Gurgel, 2018). Detected differences could in part be attributed to a variety of relevant elements such as (i) sensor limitations (and the related instrumental noise), (ii) mismatch in the horizontal sampling (whereas direction homogeneity along the HFR range cells is assumed, in situ instruments provide point measurements), and (iii) vertical mismatch (adjustment of wind measurement from 10 m a.m.s.l. to sea surface). Other physical effects such as the wind duration and fetch should also be included in the evaluation of the HFR inversion performance.
From this investigation we can draw several conclusions: (i) the inversion of wind direction is in general not reliable at low wind speeds, and (ii) additional investigations of noise interference in the returned signal with the inversion method of wind direction are still necessary.
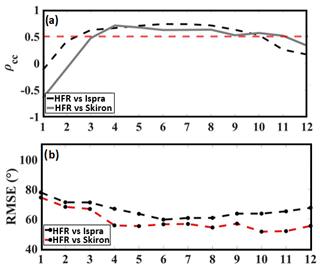
Figure 6Variation of the (a) circular correlation coefficient (ρcc) and (a) RMSE on different range cells (km from site), derived from the comparison of HFR-GoN wind direction against data provided by a weather station (ISPRA) and a numerical model (SKIRON) for the event that occurred during 6–8 February 2009 in the GoN. The red dashed line in (b) shows the circular correlation coefficient threshold (0.5), since values of ρcc>0.5 indicate a reasonable correlation between the measurements (Saviano et al., 2021).
2.3 Best practices
A key element for an effective exploitation of a large-scale HFR network, especially when operated by many different players, is the implementation of common guidelines and best practices recognized by the international community. This level of harmonization ensures that all the sites are deployed and operated with a similar and sufficient standard of quality and thereby allows researchers to assess the consistency of results when performing data analyses and applying new methodologies to different sites and geographical areas. The availability of such manuals also improves the sustainability of the HFR network, since they facilitate the exchange of operational experience between partners and help new actors to integrate their systems with reduced effort.
Harmonization of HFR systems management is also a requirement for delivering robust operational products and services. Effort has been made in Europe to review and complement existing best practices related to surface current retrievals (Mantovani et al., 2020). The Mediterranean HFR community has been actively involved in this task, especially in the framework of the EuroGOOS HFR task team and the H2020 project JERICO-NEXT, and will benefit from the progressive implementation of the defined recommendations. In this context, it is worth mentioning that the Mediterranean HFR community is also actively working with Ocean Best Practices – OBPs (https://www.oceanbestpractices.org, last access: 31 March 2022), which is a global, sustained system comprising technological solutions and community approaches to enhance management of methods as well as support the development of OBPs.
The guidelines are shaped considering the general HFR principles of operation independently from the commercial manufacturer or antenna design and setup, and they include the following:
-
site requirements for optimal HFR performance;
-
typical authorizations needed for installing and operating an HFR station;
-
list of basic accessories for ensuring protection of the equipment, remote management, and reliable data transfer;
-
items to be evaluated for estimation of deployment and operating costs;
-
key elements for a correct setup of HFR systems and suggestions for monitoring their performances;
-
scalable data management encompassing a common protocol for data processing and the standardization of a single HFR interoperable data format with a unified list of metadata descriptors; and
-
unified procedures for quality assurance–quality control (QA-QC) of HFR data in real time.
2.4 Data flow: from providers to distribution via the EU HFR Node
In 2014, EuroGOOS launched the HFR task team (Fig. 2) with the aim of promoting the implementation of an operational HFR network in Europe based on coordinated data management and integration of basic products into the major platforms for marine data distribution technology (Corgnati et al., 2021). In 2015, a pilot action coordinated by EMODnet Physics began to develop a strategy for assembling HFR metadata and data products within Europe in a uniform way to make them easily accessible and more interoperable (Fig. 2). The European Union (EU) project JERICO-NEXT (https://www.jerico-ri.eu/previous-project/jerico-next/, last access: 31 March 2022), launched in 2015, aimed to provide procedures and methodologies to enable HFR data to comply with the international standards regarding their quality and metadata, with the overall goal of integrating the European coastal observatories. In parallel, the SeaDataCloud EU project, launched in 2016, contributed to the integration and long-term preservation of historical time series from HFR into the SeaDataNet infrastructure (Fig. 2) by defining standard interoperable data and Common Data Index (CDI) metadata formats as well as quality control (QC) standard procedures for historical data. In 2016 as well, the CMEMS Service Evolution Call supported the INCREASE project, which set the basis for the integration of existing European HFR operational systems into the CMEMS-INSTAC. More recently, the EU projects JERICO-S3 and EuroSea have been continuing these efforts to further expand the standardization and interoperability of HFR data in order to promote the distribution of high-quality HFR data and improve their impact in scientific, operational, and societal applications.
The results of these integrated efforts are significant and allowed the harmonization of system requirements as well as design, data quality, and standardization of HFR data access and tools (Mantovani et al., 2020). Thanks to these achievements, the inclusion of HFR data into CMEMS-INSTAC, EMODnet Physics, and SDC Data Access was decided to ensure the improved management of several related key issues such as marine safety, marine resources, coastal and marine environment, and weather, climate, and seasonal forecast.
The EU HFR Node (Fig. 7) was established in 2018 by AZTI, CNR-ISMAR, and SOCIB under the coordination of the EuroGOOS HFR task team (Rubio et al., 2017) as the focal point and operational asset in Europe for HFR data management and dissemination (https://thredds.hfrnode.eu, last access: 31 March 2022) by promoting networking between EU infrastructures, marine data portals, and the global HFR network. The EU HFR Node has been fully operational since December 2018 to distribute tools and support for standardization to HFR providers as well as standardized near-real-time (NRT) and delayed-mode HFR radial and total current data to CMEMS-INSTAC, EMODnet Physics, and SDC Data Access. Within the European framework, the EU HFR Node is currently managing data from 16 systems (https://thredds.hfrnode.eu, last access: 31 March 2022). In particular, 5 of these 16 systems (31 %) are deployed in the Mediterranean coastline and belong to the MONGOOS network: HFR-Gibraltar, HFR-Ibiza, HFR-DeltaEbro, HFR-TirLig, and HFR-NAdr (Fig. 1). Furthermore, the EU HFR Node integrates and delivers US HFR network data to the aforementioned data portals. In particular, the EU HFR Node implements the operational chain, which encompasses data acquisition and harvesting, harmonization, formatting, QC, validation and assessment, NRT data delivery, and historical data distribution with different reprocessing levels.
The core of this service consists of the continuous development of the data model and the processing standards through discussion with operators, providers, distributors, and international experts. Based on this, the EU HFR Node maintains and updates manuals, procedure guidelines, and software tools, and it pushes them towards the HFR operators, providers, and managers via repositories and training workshops. In particular, the software tools for processing native HFR data for QC and converting them to the standard format for distribution are continuously made available to HFR operators via public GitHub repositories and releases with a DOI assigned (https://doi.org/10.5281/zenodo.2639555).
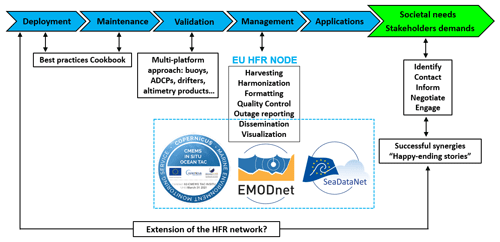
Figure 7Basic roadmap for the homogenization and distribution of HFR data from the data providers to end users.
The data processing and distribution service is founded on a simple and very effective rule (that applies only to HFR observations): if the data provider can set up the data flow according to the defined standards, the node only checks and distributes the datasets. If the data center cannot set up the data flow, the EU HFR Node directly harvests the raw data from the provider, harmonizes, quality controls, and formats these data, and makes them available to the marine data portals. The strength and flexibility of this solution reside in the architecture of the European HFR Node, which is based on a centralized database fed and updated by the operators via a webform (http://webform.hfrnode.eu, last access: 31 March 2022). The database contains updated metadata from the HFR networks as well as the needed information for processing and archiving the data. Finally, the guidelines on how to set the data flow from HFR providers to the EU HFR Node are thoroughly described in Reyes et al. (2019).
2.5 The European common data and metadata model for real-time high-frequency radar surface current data
An appropriate file description (i.e., “comprehensive metadata”), complying with accepted standards, is crucial for enforcing data discovery and access. The detailed metadata description is a prerequisite for the fully operational implementation, providing an inventory of the continuously available data for operational models. It is also necessary for providing a detailed overview of marine monitoring programs relevant for the Marine Strategy Framework Directive (MSFD) implementation.
In the framework of the aforementioned initiatives and projects, in particular within the JERICO-NEXT and INCREASE projects, a model for HFR-derived data and metadata was defined and later implemented to be the official European standard for HFR real-time data in order to ensure efficient and automated HFR data discovery and interoperability with tools and services across distributed and heterogeneous Earth science data systems.
The European HFR data format and metadata model have been defined and implemented according to the standards of the Open Geospatial Consortium (OGC) for access and delivery of geospatial data and are compliant with (i) the Climate and Forecast Metadata Convention CF-1.6, (ii) the Unidata NetCDF Attribute Convention for Data Discovery (ACDD), (iii) the OceanSITES convention, (iv) the CMEMS-INSTAC and supplemental digital content (SDC) requirements, (v) the recommendations given by the Radiowave Operators Working Group (US ROWG), and (vi) the INSPIRE (Infrastructure for spatial information in Europe) directive.
The model specifies the file format, the global attribute scheme, the dimensions, the coordinates, the data and QC variables as well as their syntax, the QC procedures, and the flagging policy. The file format is the NetCDF-4 classic model with the recommended implementation based on the community-supported CF-1.6.
Global attributes from Unidata's NetCDF Attribute Convention for Data Discovery (ACDD) are implemented, and they are divided in three categories: (i) mandatory attributes for compliance with CF-1.6 and OceanSITES conventions; (ii) recommended attributes for compliance with INSPIRE directive; and (iii) suggested attributes that can be relevant in describing the data. Attributes also have to be organized by function: discovery and identification, geospatial–temporal, conventions used, publication information, and provenance.
Variables are divided in three categories: (i) coordinate variables orienting the data in time and space (they may be dimension variables or auxiliary coordinates); (ii) data variables containing the actual measurements and information about how they were obtained; and (iii) QC variables containing the quality control flag values resulting from the QC tests performed on the data. Variable short names from SeaDataNet (SDN) P09 controlled vocabulary are recommended. CF-1.6 standard_names are required when available. The European common data and metadata model for real-time HFR surface current data are comprehensively described in the JERICO-NEXT Deliverable D5.14 (Corgnati et al., 2018).
In order to fulfill the specific requirements of CMEMS-INSTAC, EMODnet Physics, and SDC Data Access that have been operationally distributing NRT and historical HFR data since 2019, the standard data and metadata model were declined for those specific applications: the manual for the standard data and metadata model adopted in CMEMS-INSTAC and EMODnet Physics is described in Carval et al. (2020), and the one for SDC Data Access is described in Corgnati et al. (2019b).
2.6 Quality control procedures
The European common data and metadata model for real-time HFR data require a battery of QC tests in order to ensure the delivery of high-quality data and to describe in a quantitative way the accuracy of the physical information as well as to detect occasional non-realistic current vectors or artifacts (defined as spikes, spurious values, or unreliable data), generally detected at the outer edges of the HFR domain and flagged in accordance with a predefined protocol.
A battery of QC tests is consistently applied to HFR data as defined by the EuroGOOS Data Management, Exchange and Quality Work Group (DATAMEQ) recommendations on real-time QC and building on the Quality Assurance/Quality Control of Real-Time Oceanographic Data (QARTOD) manual produced by the US Integrated Ocean Observing System (IOOS). These mandatory QC tests have been selected in strict collaboration with most of the European HFR operators and data providers. While they are meant as a minimum set of QC needed for data distribution, any further QC processing of HFR data is strongly encouraged.
These standard sets of tests, which are manufacturer-independent, have been defined for both radial and total velocity data. The battery of mandatory QC tests and the flagging scheme are thoroughly described in Corgnati et al. (2018). Each QC test results in a flag related to each data vector: the flag is contained in the specific test variable. These variables can be matrices with the same dimensions of the evaluated data variable, containing, for each cell, the flag related to the vector lying in that cell in the case that the QC test evaluates each cell of the gridded data. Or they can be scalars in the case that the QC test assesses an overall property of the data file. An overall QC variable reports the quality flags related to the results of all the QC tests: it is categorized as a “good data” flag if and only if all QC tests are successfully passed by the data.
The mandatory QC tests for HFR radial velocity data are syntax, over water, variance threshold, velocity threshold, median filter, temporal derivative, average radial bearing, and radial count.
The mandatory QC tests for HFR total velocity data are data density threshold, GDOP threshold, variance threshold, velocity threshold, and temporal derivative.
However, the main drawback lies with the potential removal of accurate data when the discriminating algorithm is based on tight thresholds. Therefore, HFR operators will need to select, and keep updated, the most suitable thresholds for some of these tests. Since a successful QC effort is highly dependent upon selection of the proper thresholds, this choice cannot be done arbitrarily. Some fine-tuning, based on the specific historical conditions of the system, is thus required to have the right trade-off between confirmed outlier identification and false alarm rate, maximizing the benefit of the applications of these methods.
The Mediterranean HFR network includes 15 different systems, which cover a small portion of the entire coastal domain (Fig. 1). The limited spatial coverage is not only due to the reduced number of HFRs deployed but also to the predominant use of medium-range (13.5 MHz) and short-range (above 20 MHz) systems, whose basic technical aspects are gathered in Table 2. While these HFRs present a maximum range of 80 km, long-range systems (which operate below 5 MHz and are typically deployed in the Atlantic European waters) can map the surface circulation over broader areas for distances up to 200 km offshore (Mantovani et al., 2020). Long-range HFR systems are not deployed in the Mediterranean since they present some technical limitations in this semi-enclosed sea. On one hand, they provide surface circulation maps with coarser horizontal grid resolution (above 5 km), which are not convenient to adequately resolve some (sub)mesoscale ocean processes (i.e., eddies, instabilities) that commonly characterize the Mediterranean dynamics. On the other hand, they cannot accurately monitor the wave field under low sea states as the second-order spectrum is closer to the noise floor (and more likely to be contaminated with spurious contributions) than in the case of short- and medium-range HFR systems. Since the Mediterranean wave climate is not as intense as the Atlantic one, the use of long-range systems would result in limited precision and reduced temporal continuity in wave measurements (Lipa and Nyden, 2005). Finally, it is worth mentioning that a cross-border agreement was signed in 2018 (by Spain, France and Italy) to establish the 13–16 MHz band as the one to be used for oceanographic radars in the western Mediterranean Sea (Roarty et al., 2019).
The monitoring capabilities appear to be spatially asymmetric, with the concentration of HFR installations generally decreasing from NW to SE due to a wealth of political and socioeconomic factors. Diverse interlinked aspects influence the selection of the place to deploy such HFR systems, namely the following: (i) gaining access to suitable and unobtrusive emplacements, where electromagnetic interference (from the surrounding environment or the nearby presence of metal items, buildings, or orographic obstacles) is nonexistent or at least minimized; (ii) in the case of academia, the proximity to the research laboratory in charge of the maintenance and scientific exploitation of an HFR system (which helps to mitigate the costs of prompt recovery in the case of temporal outage); (iii) the oceanographic interest of the selected coastal area (i.e., marine protected areas, biodiversity hot spots), where ocean processes of paramount importance take place at multiple spatiotemporal scales; and (iv) the societal concern tied to the HFR location. For example, the Strait of Gibraltar (Fig. 1) constitutes a target for potential oil spill accidents due to both the extremely intense maritime traffic (as the only entrance gate to the Mediterranean Sea from the Atlantic Ocean) and the significant trade volume related to the activity of the port of the Bay of Algeciras.
Table 2Description of the HFR systems deployed in the Mediterranean Sea, which are currently working in an operational way. The list is ordered by frequency.
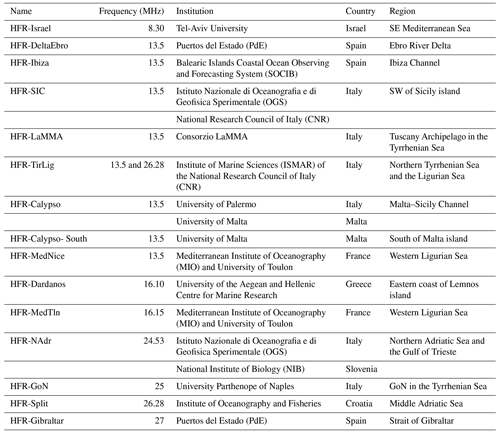
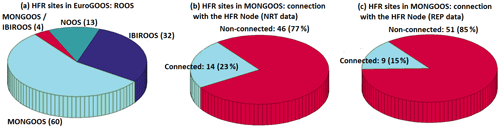
Figure 9Pie charts showing the number and percentages of HFR systems in MONGOOS in terms of Regional Operational Oceanographic Systems (ROOS) alliances and integration of near-real-time (NRT) and reprocessed (REP) HFR data into the European HFR Node (Fig. 7).
In terms of current status, the Mediterranean HFR network is characterized by the presence of a considerable number of existing sites (47), 31 of them working operationally and 16 sites out of order permanently for a variety of reasons ranging from technical to financial issues. In the short-term future, 13 new sites will be incorporated (Fig. 8a). Broadly speaking, up to 82 % of the deployments have been permanent, while a small portion of them were temporarily implemented in the framework of specific time-delimited research projects (Fig. 8b). Finally, DF HFRs are more abundant than BF systems in this regional domain (Fig. 8c).
In comparison with other regional alliances like the Iberia–Biscay–Ireland Regional Ocean Observing System (IBIROOS), MONGOOS fairly represents 55 % of the total HFR sites in Europe (Fig. 9a). Several of those MONGOOS networks (about the 23 %) are already integrated in the EU HFR Node data flow, thus providing standardized and interoperable near-real-time (NRT) datasets to the CMEMS-INSTAC, EMODnet Physics, and SDC distribution platforms (Fig. 9b). However, a smaller fraction of them (15 %) are already delivering reprocessed (REP) data (Fig. 9c). Notwithstanding, new connections are foreseen to the EU HFR Node in the coming months of 2022.
The extension and consolidation of a cross-border network of HFRs in the Mediterranean Sea, which is nowadays integrated with other existing oceanic observation infrastructures, constitute an essential process that has been supported and is still undertaken within the framework of a number of relevant cooperation projects. Some of these multi-institutional projects, which are described below, aim at building synergies among academia, management agencies, state government offices, and end users to guarantee the coordinated development of tailored products that meet societal needs, serve the marine industry with dedicated smart innovative services, and promote strategic planning and informed decision-making in the marine environment. The official logo, web link, and timeline of each project are shown in a Gantt diagram (Fig. 10).
4.1 TRADE (2010–2013)
TRADE (Trans-regional RADars for Environmental applications) was a cooperative program between Spain and Portugal (POCTEP) supported by European FEDER funding. The project's main goal was to prevent the risks associated with navigation and port operations in the SW Iberian Peninsula and the Strait of Gibraltar since this corridor has some of the most intense maritime traffic from oil and chemical tankers. To this end, an HFR system was deployed to monitor currents and waves (Lorente et al., 2014). Complementarily, a border interoperability platform was created for the management and distribution of HFR data.
4.2 TOSCA (2010–2013)
The five-country TOSCA (Tracking Oil Spills and Coastal Awareness network) pilot project was part of the MED program and supported by European Regional Development (ERD) funding. It aimed at improving the quality, speed, and effectiveness of the decision-making process in the case of marine accidents in the Mediterranean concerning oil spill pollution and SAR operations (Berta et al., 2014; Bellomo et al., 2015). Among other valuable goals, Lagrangian comparisons of HFR-derived measurements were conducted against the trajectories provided by drifters previously released in high-traffic coastal areas to provide critical information to support policy makers.
4.3 RITMARE (2012–2016)
The Italian flagship project RITMARE, funded by the Italian Ministry of University and Research, was a national research program focused on (i) the integration of the existing local observing systems toward a unified operational Italian framework and (ii) the harmonization of data collection and data management procedures (Carrara et al., 2014). A specific action was conducted for the establishment of a national coastal radar network that included both HFR and X-band radar technologies (Corgnati et al., 2014). Furthermore, a dedicated action was undertaken to foster interoperability among different data providers.
4.4 HAZADR (2013–2015)
This project, funded by the IPA Adriatic Cross-border Cooperation Programme, aimed to upgrade the knowledge framework about the estimated environmental and socioeconomic risks in the most vulnerable areas of the Adriatic Sea due to both natural and human-induced factors. Furthermore, a decision support system was implemented to track the spreading of oil spilled during hazards. The usage of six HFR systems in different applications was part of the project, with some of them (like HFR-Split, shown in Fig. 1) being installed during the project, while sharing the data in a common database.
4.5 NEURAL (2013–2015)
The main objective of NEURAL project, which was supported by the Unity Through Knowledge (UKF) Fund, was to build an efficient, reliable, and innovative prototype ocean surface current forecasting system in coastal areas of the northern Adriatic Sea by using neural network algorithms. The self-organizing map (SOM) neural network was trained jointly by the multi-year surface current fields measured by HFR and mesoscale surface winds simulated by high-resolution numerical weather prediction models. Then, based on the weather forecast and the trained SOM solutions, the prediction of surface currents was issued for the following 3 d, for which mesoscale atmospheric models have significant reliability. The SOM-based forecast was verified against an independent dataset, showing slightly higher reliability than the classical ocean forecasting system based on numerical modeling.
4.6 JERICO-NEXT (2015–2019)
The JERICO-NEXT (Joint European Research Infrastructure network for Coastal Observatory – Novel European eXpertise for coastal observaTories) initiative was developed under the Horizon 2020 (H2020) program INFRAIA. It was carried out by 33 institutions from 15 countries and emphasized that the complexity of the coastal ocean cannot be well understood if interconnection between physics, biogeochemistry, and biology is not guaranteed. Such integration required new technological developments allowing continuous monitoring of a larger set of parameters. JERICO-NEXT consisted of strengthening and enlarging a solid and transparent European network to provide operational services for a timely, continuous, and sustainable delivery of high-quality environmental data and products related to the marine environment in European coastal seas. In terms of HFR technology, the main aim was not only to harmonize data formats and best practices but also to improve current estimates (by means of advanced quality controls) to study ocean transport and connectivity between coastal and deep open-sea waters.
In this context, it is worth mentioning the ongoing JERICO-S3 and JERICO Design Study (DS) projects (2020–2023) as part of the JERICO Research Infrastructure (RI) initiative. JERICO-RI, which is a long-term integrated framework providing high-quality marine data, expertise, and multi-platform infrastructures for Europe's coastal seas, might have a significant impact in terms of integration of HFR among key coastal observing technologies.
4.7 IMPACT (2017–2020)
The IMPACT project was supported by the Interreg Italy–France maritime program. Interreg is a European territorial cooperation program designed to promote cooperation between member states on shared challenges and opportunities (https://www.interregeurope.eu/, last access: 31 March 2022). In this context, the IMPACT project aimed to establish the first transboundary HFR network between Italy and France, covering 200 km of coastline. The main goal was to define cross-border sustainable management plans to preserve marine protected areas that take into account the development needs of ports, which are both fundamental elements of the so-called blue growth. IMPACT also promoted shared best practices to improve the interoperability and usability of the entire system. IMPACT capitalized investments on HFR technology and constituted the starting point for a further expansion of the network thanks to the SICOMAR plus and SINAPSI projects, also described below.
4.8 IBISAR (2018–2020)
The IBISAR (Iberia–Biscay–Ireland Search and Rescue) service, implemented within the context of CMEMS user uptake programs, aimed at facilitating decision-making to SAR operators and emergency responders (Révelard et al., 2021; Reyes et al., 2020). IBISAR is a coastal downstream service that provides a user-friendly ocean data quality assessment with easily interpretable metrics to guide users to select the most accurate ocean forecast in the IBI region, including the western Mediterranean Sea, and facilitate decision-making. To this aim, nine ocean forecast models (four CMEMS models, two regional models, and three coastal models), six HFR systems, and all drifters available in the CMEMS catalog were integrated.
4.9 SICOMAR plus (2018–2021)
The SICOMAR plus cross-border project was supported by the Interreg Italy–France maritime program. It addressed the common challenge of navigation safety and quality of the transboundary marine environment. The project's overall objective was to reduce the risks associated with navigation accidents and their consequences for human life, goods, and the environment. It will create a coordinated system of governance tools, highly technologically innovative surveillance methods, and new safety services at sea. The project intends to launch shared strategic planning activities which will identify navigation safety solutions in high-risk marine zones of the cooperation area by setting up two joint monitoring plans for navigation and pilotage safety. The project aims to improve the coverage of monitoring networks, increase the effectiveness of risk reduction forecasting systems, enhance environmental protection services, and establish interoperable data sharing. To this end, several new HFR systems have been installed and some others upgraded along the Italian and French coastlines, respectively (Guérin et al., 2019).
4.10 CALYPSO (2011–2013), CALYPSO-FollowOn (2015), and CALYPSO-South (2018–2021)
Through the CALYPSO, CALYPSO-FollowOn, and CALYPSO-South projects (Interreg Italy–Malta maritime program), a permanent and fully operational HFR system for the real-time measurement of sea surface currents and waves in the strip of sea between Malta and Sicily was set up (Orassi et al., 2018). Data applications are opened to many different sectors, reaching out beyond research and monitoring, targeting downstream services in support of key national and regional stakeholders. The objective of the 2-year CALYPSO project (2011–2013) was the deployment of an HFR system for the permanent monitoring of the sea state. CALYPSO-FollowOn (2015) was a 6-month intensive project which built on the achievements of the CALYPSO project. It delivered a more robust HFR monitoring of sea surface currents in the Malta–Sicily Channel with the installation of an additional HFR site on the Sicilian side. CALYPSO-South (2018–2021) currently addresses the challenges of safer marine transportation, protection of human lives at sea, and safeguarding of marine and coastal resources from irreversible damage. It is a commitment to putting technological advancement and scientific endeavor at the service of humanitarian responses, reducing risks in seafaring, and protecting the marine environment. To this end, the CALYPSO HFR network coverage was expanded to the western part of the Malta–Sicily Channel and the southern approaches to the Maltese archipelago, developing new monitoring and forecasting tools as well as delivering tailored operational downstream services to assist national responsible entities in their maritime security, rescue, and emergency response commitments.
4.11 SINAPSI (2020–2022)
The SINAPSI project (Assistance to Navigation for Access to Safe Ports) is supported by the Interreg Italy–France maritime program. It aims to develop real-time tools to monitor the sea state for safe navigation and wise decision-making in port-approach areas, thereby reducing the risk of accidents. The objective will be pursued by expanding and integrating the cross-border monitoring network of traditional instruments (ADCPs, drifters, etc.) with innovative tools such as coastal HFRs. Additionally, the network will then be used to validate a series of numerical models required for the prediction of the hydrodynamic conditions in port-approach areas.
4.12 PANORAMED (2017–2022)
PANORAMED, developed under the Interreg MED program, is a governance platform that supports the process of strengthening and developing multilateral cooperation frameworks in the Mediterranean region for joint responses to common challenges. The whole Mediterranean space is represented by the 12 member states included in the partnership. Within this timeframe, PANORAMED will provide opportunities to (i) organize high-level events aiming at improving the Mediterranean area's governance covering the whole territory and (ii) promote the preparation of strategic projects through dissemination events in each country as well as the preparation and launch of the so-called “terms of reference”. During the first 2 years, PANORAMED will work on two strategic themes (coastal and maritime sustainable tourism, maritime surveillance), with a future extension of innovation as a third strategic theme.
4.13 SHAREMED (2019–2022)
SHAREMED (SHARing and Enhancing capabilities to address environmental threats in the MEDiterranean sea) is supported by the Interreg MED program. It focuses on increasing the capabilities to assess hazards related to pollution and environmental threats in Mediterranean transnational waters. This goal will be achieved by sharing knowledge, observations, and technologies as well as building common frameworks, tools, and services to evaluate the impact of environmental threats on marine ecosystems. The SHAREMED HFR group aims to enhance the quality and use of HFR observations by merging them with other observational and modeling data sources.
4.14 EuroSea (2019–2023)
The project “EuroSea: Improving and Integrating European Ocean Observing and Forecasting Systems for Sustainable use of the Oceans” is supported by the H2020 program BG2019-1. It works to enhance the European ocean observing and forecasting system in a global context by delivering ocean observations and forecasts to advance scientific knowledge about ocean climate, marine ecosystems, and their vulnerability to human impacts, thereby demonstrating the importance of the ocean for an economically viable and healthy society. It aims at advancing research and innovation towards a user-focused, truly interdisciplinary, and responsive European ocean observing and forecasting system that delivers the essential information needed for human well-being and safety, sustainable development, and a blue economy in a changing world. With regards to HFR technology, EuroSea aims to establish the governance structure (Rubio et al., 2021) and the implementation of best practices of operations, including an outage online reporting database, a standardized quality assessment, and effective data management.
4.15 iWaveNET (2020–2023)
The iWaveNET project is carried out under the Interreg Italy–Malta maritime program. It aims to implement an innovative network to monitor the sea state along the southwestern coast of Sicily in a cross-border area through the integration of different technologies encompassing HFR, directional wave buoys, high-sensitivity seismographs, tidal gauges, and numerical models. The final scope is to develop a decision support system to be transferred to interested parties (local and national authorities) for the mitigation of the coastal risk linked to extreme events (i.e., storm surges) that are potentially catastrophic in the Sicilian channel.
In conclusion, the last 10–15 years have witnessed a significant increase in national and cross-border projects in the Mediterranean Sea (Fig. 10) whose main scope was (and still is) to consolidate the HFR as an efficient coastal ocean monitoring technology. Most of the projects are funded by the European Commission in the framework of different Interreg programs, by the EU H2020 Research and Innovation, and by national research programs. In particular, 2020 has been a key year in terms of the wealth of initiatives carried out simultaneously (10). A relevant number of new HFR sites have been recently deployed and integrated into multi-platform observatories, providing quality-controlled data that are routinely delivered to a broad audience and subsequently used for diverse marine applications, including, among others, maritime safety, oil spill accidents or SAR operations (TOSCA, HAZADR, IBISAR, SICOMAR plus, CALYPSO, PANORAMED, SHAREMED, iWaveNET), port and harbor security (SINAPSI), risk prevention, and coastal management (TRADE), as well as marine spatial planning and integrated coastal zone management (RITMARE, IMPACT).
While the implementation of a fully operational HFR regional network in the Mediterranean Sea is still in progress, other observational networks have reached a very mature stage in terms of the number of permanent devices, length of recorded time series, and consistency of the quality control protocols adopted. According to Tintoré et al. (2019), there are 58 buoys capable of measuring waves (most of which are directional), 100 sea level stations, 37 operational current meters, 113 stations monitoring the seawater temperature, 50 salinity stations, and 78 Argo floats in the Mediterranean Sea. In terms of priority and significance, the HFR network might be considered a useful ancillary tool that complements in situ platforms, which nowadays constitute a sound monitoring core in this region. Special emphasis has been recently placed on other emerging technologies, such as glider facilities and biogeochemical Argo floats, thanks to their ability to monitor the three-dimensional water column. However, they are not as broadly used as HFRs, and the level of operational implementation still remains in a preliminary research phase.
5.1 General challenges
Equal to other operational ocean observing systems existing in the Mediterranean Sea (for an extensive review, see Tintoré et al., 2019), there are diverse socioeconomic and technical challenges to be tackled during the implementation of an integrated HFR regional network. A strengths–weaknesses–opportunities–threats (SWOT) analysis was performed as a situational framework to assess the current status and future prospects of this coastal network but also to evaluate the risks associated with this implementation process that could eventually help to foster long-term strategic planning and wise decision-making (Fig. 11). Among others, the top priority issue is not only the maintenance of continued financial support to preserve the infrastructure core service already implemented that is subject to costly repairs, but also the pursuit of permanent funding (thanks to Interreg programs like SICOMAR plus and CALYPSO) to extend the network at both national and regional scales for better cross-border coverage. Since local networks are frequently supported by national research funds, their long-term sustainability is often jeopardized. As a quantitative long-term objective, it would be recommended to maintain the rate reported in Rubio et al. (2017) of six new HFR sites installed per year in Europe. That might imply the installation of two to three new HFR sites per year in geostrategic coastal regions of the Mediterranean Sea such as marine protected areas, straits, or port-approach areas. To better define priority installation areas at regional level, methodological guidelines were developed in the context of the JERICO-NEXT project (Griffa et al., 2019), for which societal needs (maritime traffic density, historical SAR incidents, location of bunkering areas, biological resources, etc.) and HFR technology limitations were jointly considered. Similarly, the Mediterranean HFR network should be further implemented following these shared guidelines.
Furthermore, the monitoring capabilities are variable, with a clear north–south unbalance in the Mediterranean region for a variety of reasons. In addition to the existence of fragile and volatile political systems in southern shore countries that severely handicap sustained research programs (Fig. 11), precarious socioeconomic conditions also impact political priorities. Intermittent and uncoordinated initiatives might result in underdeveloped marine policies (at both national and regional level), significant resource dispersion, and the inefficient management of the coastal environment. In this context, the implementation of lower-cost HFRs would greatly enhance developing countries' capability to monitor coastal waters and to establish new alliances and regional partnerships. The link between MONGOOS and GOOS Africa must be strengthened in order to define common roles and shared activities in the Mediterranean Sea.
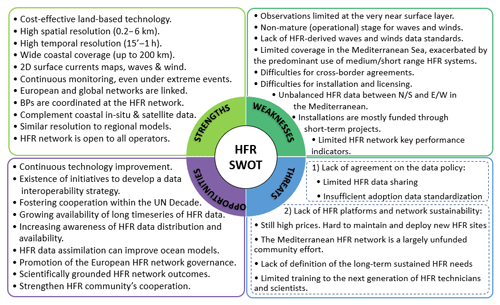
Figure 11SWOT (strengths, weaknesses, opportunities, and threats) analysis of the Mediterranean HFR network.
A network extension should fulfill a number of interlinked requirements: (i) simplification of bureaucratic processes for obtaining licenses such as source site permissions, site access, transmit licenses, and use of the data (Mantovani et al., 2020); (ii) finding and gaining access to suitable and unobtrusive emplacements; (iii) training of new technicians to operate the network, which would include the dissemination of the latest available methodologies to ensure that the most up-to-date best practices are followed; and (iv) streamlining the visibility of HFR as a non-invasive remote sensing technology for maritime surveillance with a broad range of practical applications and subsequent societal benefits. In this context, holding open-house conferences and workshops, not only focused on the HFR operator community and permitting agencies but also on a more general non-instructed audience, might be an effective way of promoting public awareness and ensuring the network's survival.
In spite of the fruitful collaborations between the HFR national networks, coordination and long-term integration at regional scale are sometimes handicapped by poor data policy and restricted data access (Fig. 11). There is still a recognized necessity for the unification of standards, the centralization of methodologies, and the documentation of best practices to increase not only the interoperability of the coastal HFR network design, operation, and maintenance tasks but also efficient data discovery (Mantovani et al., 2020). In this context, new cross-border agreements should be reached to consolidate the observing infrastructure, following the example of the one signed in 2018 by Spain, France, and Italy in the western Mediterranean Sea (Roarty et al., 2019). For instance, the monitoring of the surface circulation through the Strait of Gibraltar could be significantly enhanced thanks to a cross-border agreement between Spain and Morocco to integrate their respective HFR sites into one single network.
A complementary aspect would be the implementation of harmonized outage reporting among the HFR community at both European and Mediterranean levels. This would imply the creation of a centralized HFR outage database (Updyke, 2017) as an ancillary support tool for operations and maintenance in order to ensure HFR site sustainability (i.e., downtime, outages, and failures). It would work as a forum to share expertise, integrate approaches, and minimize the impact of temporal outages (Roarty et al., 2019).
Additionally, communication with policy makers and stakeholders is, even now, occasional and intermittent. Potential stakeholders should be clearly identified and promptly informed to boost their engagement. Coordinated actions to involve the national focal points (which are the appropriate contact points in each member state for affairs regarding the implementation of the GOOS at national and global levels) should also be performed within the European Ocean Observing System (EOOS) framework. The success of any regional alliance inexorably relies on the adoption of a win–win strategy based on transparency, with commitments that are both measurable and achievable by means of well-defined milestones. A bidirectional commitment should be built between HFR operators (“we create the tailored product you urgently need”) and stakeholders (“we will definitely use the products and services you specifically implement for us”). Afterwards, tracking and keeping commitments is recognized as one of the most relevant aspects of stakeholder relationship management. Fluent and seamless communications, tracked in a detailed and time-based manner, are essential to update all groups affected over the course of the collaboration. More importantly, both stakeholder needs and HFR operator resources can change along the commitment life span, so periodic upgrades of the action plan might be required to satisfactorily match each other. In this context, the promotion of positive synergies and success stories might constitute an effective way to attract and mobilize new stakeholders (pre-existing or new) by means of the foundational sequence “tell, sell, negotiate, enlist”. The EU HFR Node and IBISAR project (Révelard et al., 2021) constitute successful examples of this bidirectional long-term engagement between HFR operators and end users such as SASEMAR (the Spanish Marine Safety Agency). SASEMAR oversees maritime traffic control, SAR operations, marine environmental protection, and training in Spain. In this context, HFR estimations are readily ingested by the Environmental Data Server (EDS) managed by SASEMAR to enhance the emergency planning process for a prompt response.
Given the broadly accepted credibility of HFRs, this technology must be integrated into robust analysis frameworks for improved marine governance over coastal resources covering a range of dimensions, such as legislative, planning, infrastructure, technical, scientific, and institutional partnerships at the Mediterranean level. HFRs can positively contribute to the proper establishment of environmental policies and strategies, bridging the gap between research and societal challenges.
5.2 Technical challenges
The last 2 decades have witnessed the evolution of oceanographic HFR systems from a collection of local and regional instruments operated by research-oriented groups to a backbone element in emerging national coastal ocean observatories. The practical applications already developed have unequivocally demonstrated that HFR-derived surface currents are a reliable resource for SAR operations, oil spill tracking, and harmful algal bloom monitoring, among others. Additionally, pilot programs have been undertaken by national agencies to evaluate the potential ingestion of other HFR basic products such as directional wave and wind information, together with the implementation of ad hoc alert systems for tsunami detection and vessel tracking. All these scientific and operational developments have been key drivers for the steady evolution of HFR technology, which aims to respond adequately to both societal priorities and growing end-user demands.
A relevant technical challenge that must be faced and successfully overcome over the upcoming years is the resilience of HFR coastal networks, which is seriously handicapped by harsh met-ocean conditions (i.e., heat, strong wind gusts, salt, heavy rain, and moisture) and the periodic passage of storms that give rise to severe sea states (Medicanes, storm surges, and tsunamis). Serving as a recent example, the HFR system deployed in the Ebro Delta (NE Spain) was able to provide accurate and sustained observations during the record-breaking storm Gloria, which hit the NW Mediterranean Sea in January 2020, thereby proving to be resilient to extreme events (Lorente et al., 2021).
Notwithstanding, resiliency is a broad concept that applies not only to hardware, but also to software. The HF band has been described as a clutter-rich environment. HFR manufacturers have implemented and keep developing robust software in order to mitigate noise and clutter from both environmental and anthropogenic sources, including lightning, radio transmissions, ionospheric echoes, and wind turbine echoes, to name a few.
In addition to resiliency, automation in the management of HFR systems is a key element to minimize operating costs at both national and regional scales and to ensure the long-term sustainability of the network. To meet this need, HFR manufacturers include a variety of dedicated tools and software packages developed to operationally monitor radar system health in real time so abrupt anomalies in some variables (i.e., temperatures, voltage supply levels, forward and backward transmitted power, among others) or gradual degradation and failure problems can easily be detected, triggering alerts for troubleshooting. Furthermore, newly developed software, used together with information provided by the Automatic Identification System (AIS) antenna on the radar site, allows using the position of “ships of opportunity” to constantly monitor and automatically upgrade the performance of both DF and BF algorithms (Whelan et al., 2013). Despite all these available tools, HFRs do occasionally require maintenance and/or a corrective response, similar to any other observational network. However, radar operators are often purely scientifically driven and have limited capabilities and resources to cope with this, often affecting the availability and/or quality of the data obtained.
In addition, weather radar operators' footsteps should be followed since there is increasing competition for operating bandwidth. As HFR broadcast licenses were traditionally issued as secondary, obtaining dedicated frequency allocations has remained a priority for a long time. In 2012, the International Telecommunications Union (ITU) officially allocated frequency bands between 3 and 50 MHz to support HFR operations (Roarty et al., 2019). Notwithstanding, this allocation is not exclusive, especially in the Mediterranean Sea, and such bands are nowadays used by other official and non-official radio services. As previously pointed out by Bellomo et al. (2015) in the framework of the TOSCA project, acquiring a frequency allocation that allows HFR as a primary user constitutes a key objective for the Mediterranean community in order to mitigate the presence of radio frequency interference that significantly impacts HFR performance. With ITU regulations becoming increasingly adopted around the world, more and more HFR stations have to share limited, fixed frequency bands. The expansion of HFR systems in the Mediterranean makes frequency sharing and coordination among different networks of vital importance.
As a general technical challenge, HFR systems are permanently ameliorated. On one side, the hardware is steadily improved to minimize space, maintenance tasks, and inherent costs. Such improvements include (i) for DF systems, the recent development of long-range crossed-loop monopole systems on a single mast; and (ii) for phased-array BF systems, the availability of small low-cost measurement devices that allow for measuring and calibrating cable phases at the electronics rack (no fieldwork required) and the implementation of multiple input/multiple output receive antenna arrays that reduce the antenna footprint without sacrificing performance. On the other side, novel software processing strategies are constantly being developed and updated to improve the quality of the measured data. Such developments encompass the following: (i) for DF systems, new-wave processing software that considers antenna pattern measurement; and (ii) for phased-array BF systems, software upgrades to apply DF techniques to this type of HFR to improve azimuth precision on far ranges.
As a summary, the main technological challenges for the upcoming future would encompass the following: (i) improving resilience and automation to reduce operating costs, (ii) eliminating (or at least reducing) the impact of radio noise and interference through better enforcement of ITU band utilization and further development of digital filters, and (iii) increasing the technical readiness level of additional data products (beyond surface currents) via a more direct engagement with stakeholders.
5.3 Research challenges
Among the research challenges, integration must be achieved by building reinforced synergies between commercial developers, academic institutions, management agencies, and state government offices for the coordinated creation of tailored products to support end-user communities. In this context, HFR-derived products should evolve towards finer spatiotemporal scales to improve the coastal ocean monitoring, in line with the announced CMEMS coastal extension (Sánchez-Arcilla et al., 2021), and thereby adequately resolve littoral (sub)mesoscale processes of paramount relevance. The accurate retrieval of HFR surface currents remains as a top priority since it is a prerequisite for the existing applications of this shore-based technology. The main challenges, which are already being addressed to properly estimate radial velocities at increased spatial coverage, are related to the correct identification of the first-order Bragg peaks and their exact locations as well as the resolution of the received signals in range and azimuth. This would help to fulfill the recommended level of data provision: 80 % of the spatial region over 80 % of the time (Roarty et al., 2012).
Complementarily, the accurate monitoring of transport processes also remains as a prime concern due to its influence on SAR operations and oil spill emergencies. Lagrangian time-dependent approaches with HFR data, such as Lyapunov exponents (Nolan et al., 2020) and Lagrangian coherent structures (Haller, 2015), provide a robust framework to resolve coherent flow patterns. However, they are often time-consuming and computationally more expensive as they require trajectory integrations over a complete spatiotemporal velocity dataset. Since hardware or software failures in the HFR system occasionally compromise the availability of data, diverse methodologies have been proposed to fill spatiotemporal gaps in HFR measurements, encompassing self-organizing maps (SOMs), open-boundary modal analysis (OMA), and data interpolating empirical orthogonal functions (DINEOFs), among others. Despite the growing relevance of such approaches, there is still an active debate on the limits of applicability of each gap-filling method for the Lagrangian assessment of coastal ocean dynamics (Hérnandez-Carrasco et al., 2018). Nonetheless, there seems to be consensus about the convenience of combining HFR data with both Eulerian and Lagrangian approaches, when possible, to properly explore transport processes at (sub)mesoscale ranges. A halfway approach, denominated as Eulerian coherent structures, has been recently developed to connect Eulerian quantities to short-term Lagrangian transport (Serra et at., 2020), with substantial benefits in SAR operations.
HFR-derived wave parameters are receiving growing attention, but mainly within academia and research environments. In terms of operational oceanography, HFR-derived wave data are still far from being used on a near-real-time basis, in contrast to surface currents, which have reached a mature stage. In order to assess the accuracy of HFR-derived wave data, several validation studies have been carried out in the Mediterranean Sea (Table 1). Results suggest that HFR can efficiently monitor the wave field, even during extreme events when wave heights exceed the predefined saturation limit of the HFR, which depends on the frequency (Lorente et al., 2021). There are diverse challenges associated with the retrieval of wave parameters that must still be addressed to foster the operational use of this basic product, encompassing the appropriate application of a common battery of automatic checks performed in real time (to flag and subsequently filter inconsistent values or spike-like fluctuations) as well as the standardization of data and metadata structure. Additional efforts should be focused on the improvement of multiscale wave height estimation for highly variable sea states by using dual-frequency HFR systems (Wyatt and Green, 2009; Helzel et al., 2017) or by extracting wave information directly from the first-order Bragg peaks (Zhou and Wen, 2015) in order to overcome the wave height limitation at a single frequency and to better measure low and moderate waves, respectively.
Future research endeavors should also include the development of robust algorithms for a reliable measurement of wind speed, which remains less developed, that could complement the ongoing HFR multi-parameter monitoring. The limited number of studies existing in the Mediterranean areas (i.e., Ligurian Sea, Gulf of Naples) about the extraction of ocean surface wind from HFR systems seem to suggest that the accuracy of wind field inversion algorithms in coastal areas improves for higher-frequency systems under strong wind conditions. They also recommend prior knowledge about the wind field variability and climatology in the study area to better design the investigation and assess the wind field measurements.
On the other hand, the implementation of data assimilation schemes could provide an integrative framework for maximizing the joint utility of HFR-derived observations and numerical models with the aim of improving model predictive skills in coastal areas. Although a few valuable initiatives have already been carried out in the Mediterranean with positive results in the modeling of the upper-layer circulation (Hernández-Lasheras et al., 2021; Vandenbulcke et al., 2017; Marmain et al., 2014), we should further strive to develop robust, fully operational assimilation schemes for HFR data encompassing both radial and total current vectors. Equally, some previous works outside the Mediterranean study area have reported the benefits of assimilating HFR-derived wave parameters into SWAN wave models (Siddons et al., 2009) or the high-resolution coastal WAVEWATCH III model (Waters et al., 2013).
Although data assimilation is a powerful technique, advances in coastal ocean monitoring should also include an improved understanding of underlying physical processes. For instance, wave–current interactions can contribute to the generation of large-amplitude waves, which are triggered naturally when a stable wave train encounters an accelerating opposing current (Onorato et al., 2011). Ràfols et al. (2019) drew a similar conclusion via numerical simulations with coupled (hydrodynamic–wave) models in the NW Mediterranean Sea. Viitak et al. (2016) reported an increase in the wave height of up to 100 cm in nearshore waters of the eastern Baltic Sea during the St. Jude storm due to the combined effect of surface currents and sea level on the wave field evolution. In this context, HFR technology should thereby be used to effectively monitor extreme events in near-real time and unveil hydrodynamic aspects such as the aforementioned wave–current interactions (Zeng et al., 2019), which are still poorly resolved or even misrepresented by current state-of-the-art regional ocean models (Lorente et al., 2021).
Over recent decades, HFR has become commonplace in monitoring the sea state in coastal areas, once its technical capabilities and potential applications were clearly showcased. With the maturing of this technology, attention has turned to what the scientific community and other end users can learn and utilize from HFR data.
Since the Mediterranean Sea constitutes a significant geostrategic region from both commercial and oceanographic perspectives, the use of HFR has been steadily gaining recognition as an effective land-based remote sensing technology for the multi-parameter monitoring of socioeconomically vital and often environmentally stressed coastal waters. The present work is intended as a panoramic overview of the main achievements, ongoing activities, and future challenges to be faced by the Mediterranean HFR community in order to transition several stand-alone HFR systems into an integrated monitoring network operated permanently at basin scale. While the implementation of a fully operational HFR regional network in the Mediterranean Sea is still in progress and far from complete, the pragmatic lessons already learned and application examples illustrated here might be useful to similar programs under development elsewhere, such as the HFR network operating in Asia, presented at the first Ocean Radar Conference for Asia (Fujii et al., 2013). A detailed description of the roadmap adopted to transform individual radars into an integrated HFR network has been provided. To assess the maturation process into a fully operational status, the system must evolve via an implementation of phased approaches, including harmonization of HFR systems architecture, homogenization of deployment and good practices for preventive maintenance, data format convergence (i.e., standardization of file structure, metadata, and automatic quality control tests), regular validation exercises against independent in situ observations, centralization of data management and access platforms, and eventually the development of customized visualization tools and added-value products to facilitate data discovery.
The Mediterranean HFR network must face a variety of challenges for future development, most of which are shared with the European and global HFR communities. However, a few other aspects are specific to the Mediterranean Sea due to the intrinsic peculiarities of this regional semi-enclosed basin. For instance, the presence of reflections from moving ships or radio frequency interference from (non-)official radio services is more pronounced as maritime traffic is significantly intense in coastal Mediterranean areas (Bellomo et al., 2015). In this context, obtaining dedicated frequency allocation for HFR technology remains the top priority issue. A network extension to cover a substantial portion of the Mediterranean coastline also constitutes a prime concern, especially in the southern shore countries where the monitoring capabilities are extremely limited. This process is handicapped by the prominent use of medium- and short-range HFR systems which map smaller spatial domains. Moreover, the Strait of Gibraltar, the Dardanelles, and the Suez Canal (Fig. 1) act as physical constraints, leading to slow water renewal cycles and high residence times at basin scale. Consequently, global warming and the chronic degree of pollution related to anthropogenic activities impose higher pressures than in any other sea in the world, turning the Mediterranean Sea into a more vulnerable hot spot for climate change (Tuel and Eltahir, 2020).
While this paper constitutes the introductory part of a double contribution wherein the current state of the art is thoroughly presented, the second part addresses the latest scientific breakthroughs with HFR technology achieved in the Mediterranean region to fulfill stakeholder demands (Reyes et al., 2022). In particular, the second paper is built over three main cornerstones (maritime safety, extreme event monitoring, and ecological decision support) to showcase emerging research-based downstream applications of societal benefit founded on the operational use of quality-controlled HFR-derived data.
The code implemented to develop the EU HFR Node tools is available in Corgnati (2019, 2020). Equally, the code for HFR data visualization within the framework of the Copernicus Marine Service is also available in Rotllán-García (2021).
MIO's HFRToulon data, used in Sect. 2 (Fig. 3), are available at http://hfradar.univ-tln.fr/HFRADAR/squel.php?content=accueil (last access: 21 April 2022, Dumas et al., 2022a), and real-time total currents (hourly data) are available for 2020 and 2021 at https://erddap.osupytheas.fr/erddap/files/cmems_nc_cf0e_c84a_8ead/ (last access: 21 April 2022, Dumas et al., 2022b). In situ observations provided by a moored buoy (from Stazione Zoologica Anton Dohrn Napoli), used in Sect. 2 (Fig. 5), are available at https://www.szn.it/index.php/en/research/research-infrastructure-for-marine-biological-resources/access-to-marine-ecosystems-and-environmental-analysis/infrastructure-for-marine-research-irm/meda-b-napoli (last access: 21 April 2022, Zambianchi et al., 2022). In situ observations provided by a moored buoy (from Puertos del Estado), used in Sect. 2 (Figs. 4 and 5), are available at https://www.puertos.es/es-es/oceanografia/Paginas/portus.aspx (last access: 21 April 2022, de Alfonso et al., 2022).
PL and ER conceived the idea of this paper and fostered the collaboration as MONGOOS-HFR co-chairs, being in charge of overall direction and planning. All authors provided inputs for Sects. 3 and 4 and contributed to the writing of other sections, as follows. PL took the lead in writing the abstract and preparing the first draft of the document. RG and JS contributed significantly to Sects. 2 and 5. CAG, AM, MB, MBen, MM, AG, RS, DD, EZ, PF, ACE, IHC, JHL, ST, EA, CB, BD, MF, HM, VD, ML, LU, BM, and AR contributed to Sect. 2.2. YT, FC, GC, AD, and AGau contributed to Sect. 2.2.2. EZ, DC, SS, PF, and MU contributed to Sect. 2.2.2 and 2.2.3. LC and CM contributed to Sect. 2.3, 2.4, 2.5, and 2.6. Finally, JT, IV, CAG, AM, AO, LU, and VC conducted a detailed revision of the entire paper to gain cohesion.
At least one of the (co-)authors is a member of the editorial board of Ocean Science. The peer-review process was guided by an independent editor, and the authors also have no other competing interests to declare. AO and VC are guest members of the editorial board of the Special Issue from the journal. Author JS is currently employed at Qualitas Instruments Lda. Author RG currently is employed at HELZEL Messtechnik GmbH. Author PL currently is employed at NOLOGIN Consulting SL. However, these three authors have not advertised commercial products and the research has not been sponsored by any of the companies.
Publisher's note: Copernicus Publications remains neutral with regard to jurisdictional claims in published maps and institutional affiliations.
This article is part of the special issue “Advances in interdisciplinary studies at multiple scales in the Mediterranean Sea”. It is a result of the 8th MONGOOS Meeting & Workshop, Trieste, Italy, 3–5 December 2019.
This study has been partially developed in the framework of the Interreg MED Strategic Project SHAREMED, co-financed by the European Regional Development Fund under the funding program Interreg MED 2014–2020. Website: https://sharemed.interreg-med.eu/ (last access: 31 March 2022). We are also grateful for the partial support of the (i) the project PO FEAMP 2014/2020 (Misura 2.51) funded by Regione Campania (Italy), (ii) the 2017 PRIN project EMME (Exploring the fate of Mediterranean microplastic: from distribution pathways to biological effects) funded by the Italian Ministry for Research (grant agreement no. 2017WERYZP), and (iii) the CMEMS-INSTAC phase II, which provides the context of the activities for HFR data harmonization, standardization, and distribution. Collaborative discussion on data management harmonization at the European level has also been carried out thanks to the contribution of the projects INCREASE (CMEMS Service Evolution Call for Tenders 21-SE-CALL1) and SeaDataCloud (EU-H2020 GA no. 730960). It is also worth mentioning that the present work has been possible thanks to the MONGOOS collaborative network, aimed toward long-term synergies between multi-disciplinary working groups in the Mediterranean Sea in order to launch strategic initiatives and pursue funding for innovative research projects. Data were obtained from the European High-Frequency Radar Node (EU HFR NODE) Thredds Server (2022), including high-frequency radar surface current data from European and US radial sites, at https://thredds.hfrnode.eu/catalog/catalog.html (last access: 1 May 2022). Finally, the authors would like to express their gratitude to the internal reviewers, Anne Molcard, Ivica Vilibić, Charles-Antoine Guérin, and Joaquín Tintoré, as well as to the MONGOOS co-chairs, Vanessa Cardin and Alejandro Orfila, for their careful and meticulous reading of the paper. Their detailed and comprehensive reviews have been very helpful to improve the structure, reading, and finalization of the paper.
This study has been developed in the framework of the Interreg MED Strategic Project SHAREMED, co-financed by the European Regional Development Fund under the funding program Interreg MED 2014–2020 (grant agreement 6184). Website: https://sharemed.interreg-med.eu/ (last access: 21 April 2022).
This paper was edited by Anna Rubio and reviewed by Julien Mader and one anonymous referee.
Aguiar, E., Mourre, B., Juza, M., Reyes, M., Hernández-Lasheras, J., Cutolo, E., Mason, E., and Tintoré, J.: Multi-platform model assessment in the Western Mediterranean Sea: Impact of downscaling on the surface circulation and mesoscale activity, Ocean Dynam., 70, 273–288, https://doi.org/10.1007/s10236-019-01317-8, 2020.
Alattabi, Z. R., Cahl, D., and Voulgaris, G.: Swell and wind wave inversion using a single very high frequency (VHF) radar, J. Atmos. Ocean. Tech., 36, 987–1013, https://doi.org/10.1175/JTECH-D-18-0166.1, 2019.
Atwater, D. P. and Heron, M. L.: HF radar two-station baseline bisector comparisons of radial components, Proceedings of IEEE Oceans 2010, Sydney, Australia, 1–4, 2010.
Barbin, Y.: High Resolution Surface Currents Mapping using Direction Finding Method in Bistatic Radar Configuration, in Third Conference on Remote Ocean Sensing, La Spezia, Italy, 2011.
Barbin, Y., Broche P., and Forget, P.: High Resolution Azimutal Radial Current Mapping with Multisource Capability, in Radio Oceanography Workshop, Split, Croatia, 2009.
Barceló-Llull, B., Pascual, A., Ruiz, S., Escudier, R., Torner, M., and Tintoré, J.: Temporal and spatial hydrodynamic variability in the Mallorca channel (western Mediterranean Sea) from eight years of underwater glider data, J. Geophys. Res.-Oceans, 124, 2769–2786, https://doi.org/10.1029/2018JC014636, 2019.
Barrick, D. E.: First-Order Theory and Analysis of MF/HF/VHF Scatter from the Sea, IEEE T. Ant. Prop., 20, 2–10, 1972.
Barrick, D. E.: Extraction of Wave Parameters Form Measured HF Radar Sea Echoes Spectra, Radio Sci., 12, 415–424, https://doi.org/10.1029/RS012i003p00415, 1977.
Barrick, D. E.: Accuracy of parameter extraction from sample averaged sea-echo Doppler spectra, IEEE T. Ant. Prop., 28, 1–11, 1980.
Barrick, D. E., Lipa, B. J., and Crissman, R. D.: Mapping surface currents with CODAR, Sea Technol., 43–48, 1985.
Basañez, A., Lorente, P., Montero, P., Álvarez-Fanjul, E., and Pérez-Muñuzuri, V.: Quality assessment and practical interpretation of the wave parameters estimated by HF Radars in NW Spain, Remote Sens., 12, 4, 598, https://doi.org/10.3390/rs12040598, 2019.
Bellomo, L., Griffa, A., Cosoli, S., Falco, P., Gerin, R., Iermano, I., Kalampokis, A., Kokkini, Z., Lana, A., Magaldi, M. G., Mamoutos, I., Mantovani, C., Marmain, J., Potiris, E., Sayol, J. M., Barbin, Y., Berta, M., Borghini, M., Bussani, A., Corgnati, L., Dagneaux, Q., Gaggelli, J., Guterman, P., Mallarino, D., Mazzoldi, A., Molcard, A., Orfila, A., Poulain, P.-M., Quentin, C., Tintoré, J., Uttieri, M., Vetrano, A., Zambianchi, E., and Zervakis, V.: Toward an integrated HF radar network in the Mediterranean Sea to improve search and rescue and oil spill response: the TOSCA project experience, J. Oper. Oceanogr., 8, 95–107, 2015.
Berta, M., Bellomo, L., Magaldi, M. G., Griffa, A., Molcard, A., Marmain, J., Borghini, M., and Taillandier, V.: Estimating Lagrangian transport blending drifters with HF radar data and models: Results from the TOSCA experiment in the Ligurian Current (North Western Mediterranean Sea), Prog. Oceanogr., 128, 15–29, https://doi.org/10.1016/j.pocean.2014.08.004, 2014.
Campanale, C., Suaria, G., Bagnuolo, G., Baini, M., Galli, M., de Rysky, E., Ballini, M., Aliani, S., Fossi, M., and Uricchio, V.: Visual observations of floating macro litter around Italy (Mediterranean Sea), Mediterranean Mar. Sci., 20, 271–281, https://doi.org/10.12681/mms.19054, 2019.
Capodici, F., Cosoli, S., Ciraolo, G., Nasello, C., Maltese, A., Poulain, P.-M., Drago, A., Azzopardi, J., and Gauci, A.: Validation of HF radar sea surface currents in the Malta-Sicily Channel, Remote Sens. Environ., 225, 65–76, https://doi.org/10.1016/j.rse.2019.02.026, 2019.
Carrara, P., Corgnati, L., Cosoli, S., Griffa, A., Kalampokis, A., Mantovani, C., Oggioni, A., Pepe, M., Raffa, F., Serafino, F., Uttieri, M., and Zambianchi, E.: The RITMARE coastal radar network and applications to monitor marine transport infrastructures, EGU General Assembly, Wien (Austria), 16, EGU2014-14 149-1, 2014.
Carval, T., Chalkiopoulos, A., Perivoliotis, L., De Alfonso Alonso-Muñoyerro, M., Manzano Munoz, F., Ringheim, L. S., Hammarklint, T., Sotiropoulou, M., Guyot C., Rottlan, P., Corgnati, L., Marinova, V., and Jandt, S.: Copernicus Marine In Situ NetCDF format manual, Copernicus in situ TAC, https://doi.org/10.13155/59938, 2020.
Cavaleri, L., Bertotti, L., Torrisi, L., Bitner-Gregersen, E., Serio, M., and Onorato, M.: Rogue waves in crossing seas: The Louis Majesty accident, J. Geophys. Res., 117, C00J10, https://doi.org/10.1029/2012JC007923, 2012.
Chapman, R. D. and Graber, H. C.: Validation of HF radar measurements, Oceanography, 10, 76–79, 1997.
Chapman, R. D., Shay, L. K., Graber, H. C., Edson, J. B., Karachintsev, A., Trump, C. L., and Ross, D. B.: On the accuracy of HF radar surface current measurements: intercomparison with ship-based sensors, J. Geophys. Res., 102, 18737–18748, 1997.
Christaki, U., Van Wambeke, F., Lefevre, D., Lagaria, A., Prieur, L., Pujo-Pay, M., Grattepanche, J.-D., Colombet, J., Psarra, S., Dolan, J. R., Sime-Ngando, T., Conan, P., Weinbauer, M. G., and Moutin, T.: Microbial food webs and metabolic state across oligotrophic waters of the Mediterranean Sea during summer, Biogeosciences, 8, 1839–1852, https://doi.org/10.5194/bg-8-1839-2011, 2011.
Chu, X., Zhang, J., Ji, Y., Wang, Y., and Yang, L.: Extraction of wind direction from the hf hybrid sky-surface wave radar sea echoes, IEEE Aerosp. Electron. Syst. Mag., 33, 42–47, 2018.
Coll, M., Piroddi, C., Steenbeek, J., Kaschner, K., Ben Rais Lasram, F., Aguzzi, J., et al.: The biodiversity of the Mediterranean Sea: estimates, patterns, and threats, PLoS ONE, 5, e11842, https://doi.org/10.1371/journal.pone.0011842, 2010.
Corgnati, L.: LorenzoCorgnati/HFR_Node_Historical_Data_Processing: EU_HFR_NODE_Historical_Data_Processing (Version v2.1.1.6). Zenodo [code], https://doi.org/10.5281/zenodo.3569519, 2019.
Corgnati, L.: LorenzoCorgnati/HFR_Node_tools: EU_HFR_NODE_Tools (Version v2.1.2), Zenodo [code], https://doi.org/10.5281/zenodo.3855461, 2020.
Corgnati, L., Mantovani, C., Griffa, A., Berta, M., Penna, P., Celentano, P., Bellomo, L., Carlson, D.F. and D'Adamo, R.: Implementation and Validation of the ISMAR High-Frequency Coastal Radar Network in the Gulf of Manfredonia (Mediterranean Sea), IEEE J. Ocean. Eng., 44, 424–445, https://doi.org/10.1109/JOE.2018.2822518, 2019a.
Corgnati, L., Mantovani, C., Novellino, A., Jousset, S., Cramer, R. N., and Thijsse, P.: SeaDataNet data management protocols for HF Radar data, WP9 – Deliverable D9.12. Version 1.6. SeaDataNet, 83, https://doi.org/10.25607/OBP-1011, 2019b.
Corgnati, L., Carrara, P. Cosoli, S. Forneris, V. Griffa, A. Kalampokis, A. Mantovani, C., Oggioni, M. Pepe, F. Raffa, R., Santoleri, F., Serafino, S., Tronconi, Uttieri, M., and Zambianchi, E.: The RITMARE Italian coastal radar network: operational system and data interoperability framework, Proceedings of the 7th EuroGOOS Conference, Lisbon, ISBN 978-2-9601883-1-8, 2014.
Corgnati, L., Mantovani, C., Novellino, A., Rubio, A., Mader, J., Reyes, E., Griffa, A., Asensio, J. L.., Gorringe, P., Quentin, C., Breitbach, G., and Widera, J.: Recommendation Report 2 on improved common procedures for HFR QC analysis, http://www.jerico-ri.eu/download/jerico-next-deliverables/JERICO-NEXT-Deliverable_5.14_V1.pdf (last access: 31 March 2022), 2018.
Corgnati, L., Mantovani, C., Rubio, A., Reyes, E., Rotllán, P., Novellino, A., Gorringe, P., Solabarrieta, L., Griffa, A. and and Mader, J: The EuroGOOS High Frequency Radar Task Team: a success story of collaboration to be kept alive and made growing, 9th EuroGOOS International conference, Shom; Ifremer; EuroGOOS AISBL, May 2021, Brest, France, 467–474, 2021.
Cosoli, S., Mazzoldi, A., and Gacic, M.: Validation of surface current measurements in the Northern Adriatic Sea from High Frequency radars, J. Atmos. Ocean. Tech., 27, 908–919, 2010.
Cotroneo, Y., Aulicino, G., Ruiz, S., Sánchez Román, A., Torner Tomàs, M., Pascual, A., Fusco, G., Heslop, E., Tintoré, J., and Budillon, G.: Glider data collected during the Algerian Basin Circulation Unmanned Survey, Earth Syst. Sci. Data, 11, 147–161, https://doi.org/10.5194/essd-11-147-2019, 2019.
Crombie, D. D.: Doppler Spectrum of Sea Echo at 13.56 Mc./s., Nature, 175, 681–682, 1955.
Davis, R. E.: Drifter observations of coastal surface currents during CODE: The method and descriptive view, J. Geophys. Res., 90, 4741–4755, https://doi.org/10.1029/JC090iC03p04741, 1985.
de Alfonso, M., Ruiz Gil-de la Serna, M. I., and Pérez, S.: Banco de datos oceanográficos, conjunto de datos REDEXT, Puertos del Estado, [data set], https://www.puertos.es/es-es/oceanografia/Paginas/portus.aspx, last access: 21 April 2022
de Paolo, T., Terril, E., and Kirincich, A.: Improving SeaSonde radial velocity accuracy and variance using radial metrics, IEEE Oceans, Genova, 1–9, 2015.
de Valk, C., Reniers, A., Atanga, J., Vizinho, A., and Vogelzang, J.: Monitoring surface waves in coastal waters by integrating HF radar measurement and modelling, Coast. Eng., 37, 431–453, https://doi.org/10.1016/S0378-3839(99)00037-X, 1999.
Dobricic, S. and Pinardi, N.: An oceanographic three-dimensional variational data assimilation scheme, Ocean Model, 22, 89–105, 2008.
Domps, B., Marmain, J. and Guérin, C.-A.: A Reanalysis of the October 2016 “Meteotsunami” in British Columbia with Help of High-Frequency Radars and Autoregressive Modeling, IEEE T. Geosci. Remote, 19, 3505105 https://doi.org/10.1109/LGRS.2021.3066849, 2020.
Domps, B., Dumas, D. Guérin, C.-A., and Marmain, J.: High-Frequency Radar Ocean Current Mapping at Rapid Scale with Auto-Regressive Modeling, IEEE J. Ocean Eng., 46, 891–899, https://doi.org/10.1109/JOE.2020.3048507, 2021.
D'Ortenzio, F., Taillandier, V., Claustre, H., Prieur, L. M., Leymarie, E., Mignot, A., Poteau, A., Penkerc'h, C., and Schmechtig, C. M.: Biogeochemical Argo: The Test Case of the NAOS Mediterranean Array, Front. Mar. Sci., 24, https://doi.org/10.3389/fmars.2020.00120, 2020.
Dumas, D. and Guérin, C.-A.: Self-calibration and antenna grouping for bistatic oceanographic High-Frequency Radars, Electr. Eng. Sys. Sci., 1–16, https://doi.org/10.48550/arXiv.2005.10528, 2020.
Dumas, D., Gramoullé, A., Guérin, C.-A., Molcard, A., Ourmiëres, Y., and Zakardjian, B.: Multistatic estimation of high-frequency radar surface currents in the region of Toulon, Ocean Dynam., 70, 1485–1503, https://doi.org/10.1007/s10236-020-01406-z, 2020.
Dumas, D., Guérin, C.-A., Quentin, C., and Molcard, A.: MIO's HFRToulon data, Mediterranean Institute of Oceanography, [data set], http://hfradar.univ-tln.fr/HFRADAR/squel.php?content=accueil, last access: 21 April 2022a.
Dumas, D., Quentin, C., and Guérin, C.-A.: Real time total currents foe 2020 and 2021, Mediterranean Institute of Oceanography, [data set], https://erddap.osupytheas.fr/erddap/files/cmems_nc_cf0e_c84a_8ead/, last access: 21 April 2022b.
Emery, B. M.: Evaluation of alternative direction-of-arrival methods for oceanographic HF radars, IEEE J. Ocean. Eng., 45, 990–1003, https://doi.org/10.1109/JOE.2019.2914537, 2020.
Emery, B. M., Washburn, M. L., and Harlan, J. A.: Evaluating radial current measurements from CODAR high frequency radars with moored current meters, J. Atmos. Ocean. Tech., 21, 1259–1271, 2004.
Esposito, G., Saviano S., Di Lemma, R., Buonocore, B., de Ruggiero, P., Pierini, S., and Zambianchi, E.: An analysis of the seasonal wind regime in the Gulf of Naples using observational data and model outputs, Geophys. Res. Abstr., 18, 13421, 2018.
Falco, P., Buonocore, B., Cianelli, D., De Luca, L., Giordano, A., Iermano, I., Kalampokis, A., Saviano, S., Uttieri, M., Zambardino, G., and Zambianchi, E.: Dynamics and sea state in the Gulf of Naples: potential use of high-frequency radar data in an operational oceanographic context, J. Oper. Oceanogr., 9, 33–45, https://doi.org/10.1080/1755876X.2015.1115633, 2016.
Fichaut, M. and Schaap, D.: From SeaDataNet II to SeaDataCloud, in International conference on Marine Data and Information systems (IMDIS), Gdansk, Poland, 57, 11–13, 2016.
Flores-Vidal, X., Flament, P., Durazo, R., Chavanne, C., and Gurgel, K.-W.: High-frequency radars: Beamforming calibrations using ships as reflectors, J. Atmos. Ocean. Tech., 30, 638–648, 2013.
Forney, R., Roarty, H., and Glenn, S.: Measuring Waves with a Compact HF Radar, Paper presented at OCEANS 2015 – MTS/IEEE, Washington, 19–22 October 2015.
Fujii, S., Heron, M. L., Kim, K., Lai, J. W., Lee, S. H., Wu, X., Wyatt, L. R., and Yang, W. C: An overview of developments and applications of oceanographic radar networks in Asia and oceania countries, Ocean Sci. J., 48, 69–97, 2013.
Gaiser, L. and Hribar, D.: Euro-Mediterranean Region: Resurged Geopolitical Importance, Int. J. Euro-Mediter. Stud., 5, 57–69, https://doi.org/10.1007/s40321-012-0002-8, 2012.
Garrabou, J., Gómez-Gras, D., Ledoux, J.-B., Linares, C., et al.: Collaborative Database to Track Mass Mortality Events in the Mediterranean Sea, Front. Mar. Sci., 6, 707, https://doi.org/10.3389/fmars.2019.00707, 2019.
Gómez, R., Helzel, T., Wyatt, L., López, G., Conley, D., Thomas, N., Smet, S., and Sicot, G.: Estimation of Wave Parameters from HF Radar Using Different Methodologies and Compared with Wave Buoy Measurements at the Wave Hub, Conference paper presented at OCEANS 2015 – MTS/IEEE, Genova, 18–21 May 2015.
Gómez, R., Tran, T. H., Ramdhani, A., and Triyono, R.: HF Radar Validation and Accuracy Analysis using Baseline Comparison Approach in the Sunda Strait, Technical Report, https://doi.org/10.13140/RG.2.2.11110.96322, 2020.
Gómez-Navarro, L., Fablet, R., Mason, E., Pascual, A., Mourre, B., Cosme, E., and Le Sommer, J.: SWOT Spatial Scales in the Western Mediterranean Sea Derived from Pseudo-Observations and an Ad Hoc Filtering, Remote Sens., 10, 599, https://doi.org/10.3390/rs10040599, 2018.
Griffa, A., Horstmann, J., Mader, J., Rubio, A., Berta, M., Orfila, A., and Axell, L.: Report on final assessment of methodological improvements and testing, JERICO-NEXT WP3 Innovations in Technology and Methodology, Deliverable D3.4, Version 2, Brest, France, IFREMER, 56 pp., (JERICO-NEXT-WP3-D3.4-180719-V2), https://doi.org/10.25607/OBP-948, 2019.
Guérin, C.-A., Dumas, S., Gramoulle, A., Quentin, C., Saillard, M., and Molcard, A.: The multistatic oceanographic HF radar network in Toulon. 2019 International Radar Conference (RADAR), Toulon, France, 2019, 1–5, https://doi.org/10.1109/RADAR41533.2019.171401, 2019.
Guérin, C.-A., Dumas, D., Molcard, A., Quentin, C., Zakardjian, B., Gramoullé, A., and Berta, M.: High-Frequency radar measurements with CODARs in the region of Nice: improved calibration and performances, accepted, J. Atmos. Ocean. Tech., 38, 2003–2016, https://doi.org/10.1175/JTECH-D-21-0058.1, 2021.
Gurgel, K. W., Antonischski, G., Essen, H. H., and Schlick, T.: Wellen radar (WERA): A new ground wave radar for remote sensing, Coast. Eng., 37, 219–234, 1999.
Gurgel, K. W., Essen, H. H., and Schlick, T.: An empirical method to derive ocean waves from second-order Bragg scattering: Prospects and limitations, IEEE J. Ocean. Eng., 31, 804–811, 2006.
Gurgel, K.-W., Barbin, Y., and Schlick, T.: Radio Frequency Interference Suppression Techniques in FMCW Modulated HF Radars, in Oceans 2007 Europe, 1–4, https://doi.org/10.1109/OCEANSE.2007.4302289, 2007.
Haller, G.: Lagrangian coherent structures, Annu. Rev. Fluid Mech, 47, 137–162, https://doi.org/10.1146/annurev-fluid-010313-141322, 2015.
Hernández-Carrasco, I., Solabarrieta, L., Rubio, A., Esnaola, G., Reyes, E., and Orfila, A.: Impact of HF radar current gap-filling methodologies on the Lagrangian assessment of coastal dynamics, Ocean Sci., 14, 827–847, https://doi.org/10.5194/os-14-827-2018, 2018.
Hernandez-Lasheras, J., Mourre, B., Orfila, A., Santana, A., Reyes, E., and Tintoré, J.: Evaluating high-frequency radar data assimilation impact in coastal ocean operational modelling, Ocean Sci., 17, 1157–1175, https://doi.org/10.5194/os-17-1157-2021, 2021.
Helzel, T., Petersen, L., Widera, J., Bennis, A., Benoit, L., and Barbin, Y.: Dual frequency ocean radar concept to measure ocean currents and waves at the Raz Blanchard, OCEANS 2017, Aberdeen, 1–4, https://doi.org/10.1109/OCEANSE.2017.8084761, 2017.
Heron, M. L., and Rose, R.: On the application of HF ocean radar to the observation of temporal and spatial changes in wind direction, IEEE J. Ocean. Eng., 11, 210–218, https://doi.org/10.1109/JOE.1986.1145173, 1986.
Heron, M. L.: Applying a unified directional wave spectrum to the remote sensing of wind wave directional spreading, Can. J. Remote. Sens, 28, 346–353, 2002.
Heron, M. L., Dzvonkovskaya, A., and Helzel, T.: HF radar optimised for tsunami monitoring, Proc. OCEANS 2015 – Genova, 1–5, 2015.
Hisaki, Y.: Nonlinear inversion of the integral equation to estimate ocean wave spectra from HF radar, Radio Sci., 31, 25–39, https://doi.org/10.1029/95RS02439, 1996.
Hisaki, Y.: Ocean Wave Directional Spectra Estimation from an HF Ocean Radar with a Single Antenna Array: Methodology, J. Atmos. Ocean. Tech., 23, 268–286, https://doi.org/10.1175/JTECH1836.1., 2006.
Hisaki, Y.: Sea Surface Wind Correction Using HF Ocean Radar and Its Impact on Coastal Wave Prediction, J. Atmos. Ocean. Technol., 34, 2001–2020, 2017.
Huang, W., Gill, E., Wu, S., Wen, B., Yang, Z., and Hou, J.: Measuring surface wind direction by monostatic HF ground-wave radar at the eastern China sea, IEEE J. Ocean. Eng., 29, 1032–1037, 2004.
Juza, M. and Tintoré, J.: Multivariate Sub-Regional Ocean Indicators in the Mediterranean Sea: From Event Detection to Climate Change Estimations, Front. Mar. Sci., 8, 610589, https://doi.org/10.3389/fmars.2021.610589, 2021.
Kalampokis, A., Uttieri, M., Poulain, P., and Zambianchi, E.: Validation of HF Radar-Derived Currents in the Gulf of Naples With Lagrangian Data, IEEE Geosci. Remote Sens. Lett., 13, 1452–1456, https://doi.org/10.1109/LGRS.2016.2591258, 2016.
Kassis, D. and Korres, G.: Hydrography of the Eastern Mediterranean basin derived from argo floats profile data, Deep Sea Res. Pt. II, 171, 104712, https://doi.org/10.1016/j.dsr2.2019.104712, 2020.
Kirincich, A.: Remote Sensing of the Surface Wind Field over the Coastal Ocean via Direct Calibration of HF radar Backscatter Power, J. Atmos. Ocean. Technol., 33, 1377–1392, 2016.
Kirincich, A. R., de Paolo, T., and Terrill, E.: Improving HF Radar Estimates of Surface Currents Using Signal Quality Metrics, with Application to the MVCO High-Resolution Radar System, J. Atmos. Ocean. Tech., 29, 1377–1390, 2012.
Kohut, J. T. and Glenn, S. M.: Improving HF radar surface current measurements with measured antenna beam patterns, J. Atmos. Ocean. Tech., 20, 1303–1316, 2003.
Kohut, J. T., Roarty, H. J., and Glenn, S.: Characterizing Observed Environmental variability with HF Doppler radar surface mappers and Acoustic Doppler Current Profilers: Environmental variability in the Coastal Ocean, J. Ocean. Eng., 31, 876–884, 2006.
Lana, A., Marmain, J., Fernández, V., Tintoré, J., and Orfilia, A.: Wind influence on surface current variability in the Ibiza Channel from HF Radar, Ocean Dynam., 66, 483–497, 2016.
Laws, K. E., Paduan, J. D., and Fernández, D. M.: Effect of Stokes drift on HF radar measurements, Radiowave Oceanography the First International Workshop, University of Miami, Rosentiel School of Marine and Atmospheric Science, 49–55, 2003.
Lejeusne, C., Chevaldonne, P., Pergent-Martini, C., Boudouresque, C. F., and Perez, T.: Climate change effects on a miniature ocean: the highly diverse, highly impacted Mediterranean Sea, Trends Ecol. Evol., 25, 250–260, https://doi.org/10.1016/j.tree.2009.10.009, 2010.
Le Traon, P. Y.: From satellite altimetry to Argo and operational oceanography: three revolutions in oceanography, Ocean Sci., 9, 901–915, https://doi.org/10.5194/os-9-901-2013, 2013.
Le Traon, P.-Y., Ali, A., Álvarez Fanjul, E., Behrens, A., Stanev, E., Staneva, J., et al.: The Copernicus Marine Environmental Monitoring Service: Main Scientific Achievements and Future Prospects, Special Issue Mercator Oceìan Journal, 56, https://doi.org/10.25575/56, 2017.
Linnenluecke, M. K., Griffiths, A., and Winn, M.: Extreme Weather Events and the Critical Importance of Anticipatory Adaptation and Organizational Resilience in Responding to Impacts, Business Strategy and the Environment, 21, 17–32, https://doi.org/10.1002/bse.708, 2012.
Lipa, B. J. and Nyden, B.: Directional wave information from the SeaSonde, IEEE J. Ocean. Eng., 30, 221–231, 2005.
Lipa, B. J., Nyden, B., Barrick, D., and Kohut, J.: HF Radar Sea-Echo from Shallow Water, Sensors, 8, 4611–4635, 2008.
Lipa, B. J., Isaccson, J., Nyden, B., and Barrick, D.: Tsunami Arrival Detection with High Frequency (HF) Radar, Remote Sens., 4, 1448–1461, 2012.
Lipa, B. J., Barrick, D., Alonso-Martirena, A., Fernandes, M., Ferrer, M. I., and Nyden, B.: Brahan Project High Frequency Radar Ocean Measurements: Currents, Winds, Waves and Their Interactions, Remote Sens, 6, 12094–12117, https://doi.org/10.3390/rs61212094, 2014.
Long, A. E. and Trizna, D. B.: Measurements and preliminary interpretation of hf radar Doppler spectra from the sea-echo of an atlantic storm, NRL Rep., 21, 680–685, 1972.
Long, R. M., Barrick, D., Largier, J. L., and Garfield, N.: Wave Observations from Central California: SeaSonde Systems and in Situ Wave Measurements, J. Sensors, 2011, 728936, https://doi.org/10.1155/2011/728936, 2011.
López, G., Conley, D., and Greaves, D.: Calibration, Validation and Analysis if an Empirical Algorithm for the Retrieval of Wave Spectra from HF Radar Sea Echo, J. Atmos. Ocean. Tech., 33, 245–261, https://doi.org/10.1175/JTECH-D-15-0159.1, 2016.
López, G. and Conley, D. C.: Comparison of HF Radar Fields of Directional Wave Spectra Against In Situ Measurements at Multiple Locations, J. Mar. Sci. Eng., 7, 271, https://doi.org/10.3390/jmse7080271, 2019.
Lorente, P., Piedracoba, S., Soto-Navarro, J., and Álvarez-Fanjul, E.: Accuracy assessment of High Frequency radar current measurements in the Strait of Gibraltar, J. Operat. Oceanogr., 7, 59–73, 2014.
Lorente, P., Piedracoba, S., Soto-Navarro, J., and Alvarez-Fanjul, E.: Evaluating the surface circulation in the Ebro delta (northeastern Spain) with quality-controlled high-frequency radar measurements, Ocean Sci., 11, 921–935, https://doi.org/10.5194/os-11-921-2015, 2015.
Lorente, P., Piedracoba, S., Soto-Navarro, J., and Álvarez-Fanjul, E.: Characterizing the surface circulation in Ebro Delta (NW Mediterranean) with HF radar and modeled current data, J. Mar. Syst., 163, 61–79, 2016a.
Lorente, P., Piedracoba, S., Sotillo M. G., Aznar, R., Amo-Baladrón, A., Pascual, A., Soto-Navarro, J., and Álvarez-Fanjul, E.: Ocean model skill assessment in the NW Mediterranean using multi-sensor data, J. Oper. Oceanogr., 9, 75–92, https://doi.org/10.1080/1755876X.2016.1215224, 2016b.
Lorente, P., García-Sotillo, M., Amo-Baladrón, A., Aznar, R., Levier, B., Sánchez-Garrido, J. C., Sammartino, S., de Pascual-Collar, Á., Reffray, G., Toledano, C., and Álvarez-Fanjul, E.: Skill assessment of global, regional, and coastal circulation forecast models: evaluating the benefits of dynamical downscaling in IBI (Iberia–Biscay–Ireland) surface waters, Ocean Sci., 15, 967–996, https://doi.org/10.5194/os-15-967-2019, 2019.
Lorente, P., Lin-Ye, J., García-León, M., Reyes, E., Fernandes, M., Sotillo, M.G., Espino, M., Ruiz, M. I., Gracia, V., Pérez, S., Aznar, R., Alonso-Martirena, A., and Álvarez-Fanjul, E.: On the Performance of High Frequency Radar in the Western Mediterranean During the Record-Breaking Storm Gloria, Front. Mar. Sci., 8, 645762, https://doi.org/10.3389/fmars.2021.645762, 2021.
Mahadevan, A., D'Asaro, E., Allen, J., et al.: CALYPSO 2019 Cruise Report: Field Campaign in the Mediterranean, Technical Report, Woods Hole Oceanographic Institution, https://doi.org/10.1575/1912/25266, 2020.
Malanotte-Rizzoli, P., Artale, V., Borzelli-Eusebi, G. L., Brenner, S., Crise, A., Gacic, M., Kress, N., Marullo, S., Ribera d'Alcalà, M., Sofianos, S., Tanhua, T., Theocharis, A., Alvarez, M., Ashkenazy, Y., Bergamasco, A., Cardin, V., Carniel, S., Civitarese, G., D'Ortenzio, F., Font, J., Garcia-Ladona, E., Garcia-Lafuente, J. M., Gogou, A., Gregoire, M., Hainbucher, D., Kontoyannis, H., Kovacevic, V., Kraskapoulou, E., Kroskos, G., Incarbona, A., Mazzocchi, M. G., Orlic, M., Ozsoy, E., Pascual, A., Poulain, P.-M., Roether, W., Rubino, A., Schroeder, K., Siokou-Frangou, J., Souvermezoglou, E., Sprovieri, M., Tintoré, J., and Triantafyllou, G.: Physical forcing and physical/biochemical variability of the Mediterranean Sea: a review of unresolved issues and directions for future research, Ocean Sci., 10, 281–322, https://doi.org/10.5194/os-10-281-2014, 2014.
Mantovani, C., Corgnati, L., Horstmann, J., Rubio, A., Reyes, E., Quentin, C., Cosoli, S., Asensio, J. L., Mader, J., and Griffa, A.: Best Practices on High Frequency Radar Deployment and Operation for Ocean Current Measurement, Front. Mar. Sci., 7, 210, https://doi.org/10.3389/fmars.2020.00210, 2020.
Marmain, J., Molcard, A., Forget, P., Barth, A., and Ourmières, Y.: Assimilation of HF radar surface currents to optimize forcing in the northwestern Mediterranean Sea, Nonlin. Processes Geophys., 21, 659–675, https://doi.org/10.5194/npg-21-659-2014, 2014.
Martín Miguez, B., Novelino, A., Vinci, M., Claus, S., Calewaert, J.-B., Valius, H., et al.: The European Marine Observation and Data Network (EMODnet): visions and roles of the gateway to marine data in Europe, Front. Mar. Sci., 6, 313, https://doi.org/10.3389/fmars.2019.00313, 2019.
Melet, A., Teatini, P., Le Cozannet, G., Jamet, C., Conversi, A., Benveniste, J., and Almar, R.: Earth Observations for Monitoring Marine Coastal Hazards and their drivers, Surv. Geophys., 41, 1489–1534, 2020.
Milglietta, M. M. and Rotunno, R.: Development mechanisms for Mediterranean tropical-like cyclones (medicanes), Q. J. Roy. Meteorol. Soc., 145, 1444–1460, 2019.
Millot, C. and Taupier-Letage, I.: Circulation in the Mediterranean Sea, edited by: Saliot, A., The Mediterranean Sea, Handbook of Environmental Chemistry, 5K, Springer, Berlin, Heidelberg, https://doi.org/10.1007/b107143, 2005.
Molcard, A., Poulain, P., Forget, P., Griffa, A., Barbin, Y., Gaggelli, J., Maistre, J. D., and Rixen, M.: Comparison between vhf radar observations and data from drifter clusters in the Gulf of la Spezia (Mediterranean Sea), J. Mar. Sys., 78, 79–89, https://doi.org/10.1016/j.jmarsys.2009.01.012, 2009.
Moltmann, T., Turton, J., Zhang, H-M., Nolan, G., Gouldman, C., Griesbauer, L., Willis, Z., Piniella, Á.M., Barrell, S., Andersson, E., Gallage, C., Charpentier, E., Belbeoch, M., Poli, P., Rea, A., Burger, E.F., Legler, D.M., Lumpkin, R., Meinig, C., O'Brien, K., Saha, K., Sutton, A., Zhang, D., and Zhang, Y.: A Global Ocean Observing System (GOOS), delivered through enhanced collaboration across regions, communities, and new technologies, Front. Mar. Sci., 6, 291, https://doi.org/10.3389/fmars.2019.00291, 2019.
Mourre, B., Aguiar, E., Juza, M., Hernández-Lasheras, J., Reyes, E., Heslop, E., Escudier, R., Cutolo, E., Ruiz, S., Mason, E., Pascual, A., and Tintoré, J.: Assessment of high-resolution regional ocean prediction systems using muli-platform observations: illustrations in the Western Mediterranean Sea, in: “New Frontiers in Operational Oceanography”, edited by: Chassignet, E., Pascual, A., Tintoré, J., and Verron, J., GODAE Ocean View, 663–694, https://doi.org/10.17125/gov2018.ch24, 2018.
Nikolaidis, N. P., Bidoglio, G., Bouraoui, F., and Cardoso, A. C.: Water Quality of the Mediterranean, Comprehensive Water Quality and Purification, 4, 230–250, https://doi.org/10.1016/B978-0-12-382182-9.00076-1, 2014.
Nolan, P. J., Serra, M., and Ross, S. D.: Finite-time Lyapunov exponents in the instantaneous limit and material transport, Nonlinear Dyn., 100, 3825–3852, https://doi.org/10.1007/s11071-020-05713-4, 2020.
Ohlmann, C., White, P., Washburn, L., Terril, E., Emery, B., and Otero, M.: Interpretation of coastal HF radar-derived currents with high-resolution drifter data, J. Atmos. Ocean. Tech., 24, 666–680, 2007.
Olmedo, E., Taupier-Letage, I., Turiel, A., and Alvera-Azcárate, A.: Improving SMOS Sea Surface Salinity in the Western Mediterranean Sea through Multivariate and Multifractal Analysis, Remote Sens., 10, 485, https://doi.org/10.3390/rs10030485, 2018.
Onorato, M., Proment, D., and Toffoli, A.: Triggering Rogue Waves in Opposing Currents, Phys. Rev. Lett. 107, 184502, https://doi.org/10.1103/PhysRevLett.107.184502, 2011.
Orasi, A., Picone, M., Drago, A., Capodici, F., Gauci, A., Nardone, G., Inghilesi, R., Azzopardi, J., Galea, A., Ciraolo, G., and Musulin, J. S.; Alonso-Martirena, A.: HF Radar for Wind Waves Measurements in the Malta-Sicily Channel, Measurements, 128, 446–454, 2018.
Paduan, J. D., Kim, K. C., Cook, M. S., and Chavez, F. P.: Calibration and validation of direction-finding High-Frequency radar ocean surface current observations, IEEE J. Ocean. Eng., 31, 862–875, 2006.
Pinardi, N., Arneri, E., Crise, A., Ravaioli, M., and Zavatarelli, M.: The physical, sedimentary and ecological structure and variability of shelf areas in the Mediterranean Sea, in: The Sea, 14, edited by: Robinson, A. R. and Brink, K., Cambridge, Harvard University Press, 1243–1330, 2006.
Pinardi, N., Cessi, P., Borile, F., and Wolfe, W. C.: The Mediterranean Sea overturning circulation, J. Phys. Oceanogr., 49, 1699–1721, https://doi.org/10.1175/JPO-D-18-0254.1, 2019.
Ràfols, L., Grifoll, M., and Espino, M.: Wave–current interactions in a wind-jet region, Ocean Sci., 15, 1–20, https://doi.org/10.5194/os-15-1-2019, 2019.
Ramos, R. J., Graber, H. C., and Haus, B. K: Observation of Wave Energy Evolution in Coastal Areas Using HF Radar, J. Atmos. Ocean. Tech., 26, 1891–1909, https://doi.org/10.1175/2009JTECHO631.1, 2009.
Ranalli, M., Lagona, F., Picone, M., and Zambianchi, E.: Segmentation of sea current fields by cylindrical hidden Markov models: a composite likelihood approach, J. Roy. Statist. Soc., 67, 575–598, 2018.
Révelard, A., Reyes, E., Mourre, B., Hernández-Carrasco, I., Rubio, A., Lorente, P., Fernández, C. D., Mader, J., Álvarez-Fanjul, E., and Tintoré, J.: Sensitivity of Skill Score metric to validate Lagrangian simulations in coastal areas: recommendations for Search and Rescue applications, Front. Mar. Sci., https://doi.org/10.3389/fmars.2021.630388, 2021.
Reyes, E., Rotllán-García, P., Rubio, A., Corgnati, L., Mader, J., and Mantovani, C.: Guidelines on how to sync your High Frequency (HF) radar data with the European HF Radar node (Version 1.2), Balearic Islands Coastal Observing and Forecasting System, SOCIB, https://doi.org/10.25704/9XPF-76G7, 2019.
Reyes, E., Hernández-Carrasco, I., Révelard, A., Mourre, B., Rotllán, P., Comerma, E., Bakhsh, T. T., Rubio, A., Mader, J., Ferrer, L., De Lera Fernández, C., Álvarez-Fanjul, E., and Tintoré, J.: IBISAR service for real-time data ranking in the IBI area for emergency responders and SAR operators, in: Copernicus Marine Service Ocean State Report, Issue 4, J. Oper. Oceanogr., 13, 92–99, https://doi.org/10.1080/1755876X.2020.1785097, 2020.
Reyes, E., Aguiar, E., Bendoni, M., Berta, M., Brandini, C., Cáceres-Euse, A., Capodici, F., Cardin, V., Cianelli, D., Ciraolo, G., Corgnati, L., Dadić, V., Doronzo, B., Drago, A., Dumas, D., Falco, P., Fattorini, M., Fernández, M. J., Gauci, A., Gómez, R., Griffa, A., Guérin, C.-A., Hernández-Carrasco, I., Hernández-Lasheras, J., Ličer, M., Lorente, P., Magaldi, M. G., Mantovani, C., Mihanović, H., Molcard, A., Mourre, B., Révelard, A., Reyes-Suárez, C., Saviano, S., Sciascia, R., Taddei, S., Tintoré, J., Toledo, Y., Uttieri, M., Vilibić, I., Zambianchi, E., and Orfila, A.: Coastal high-frequency radars in the Mediterranean – Part 2: Applications in support of science priorities and societal needs, Ocean Sci., 18, 797–837, https://doi.org/10.5194/os-18-797-2022, 2022.
Roarty, H., Smith, M., Kerfoot, J., Kohut, J., and Glenn, S.: Automated quality control of high frequency radar data, IEEE Oceans, 2012, 1–7, 2012.
Roarty, H., Smith, M., Glenn, S. M., Barrick, D. E., Page, E., Statscewich, H., and Weingartner, T.: Expanding Maritime Domain Awareness Capabilities in the Arctic: High Frequency Radar Vessel-tracking, IEEE Radar Conference, 1–5, 2013.
Roarty, H., Cook, T., Hazard, L., Harlan, J., Cosoli, S., Wyatt, L., Fanjul, E. A., Terrill, E., Otero, M., Largier, J., Glenn, S., Ebuchi, N., Whitehouse, B., Bartlett, K., Mader, J., Rubio, A., Corgnati, L. P., Mantovani, C., Griffa, A., Reyes, E., Lorente, P., Flores-Vidal, X., Rogowski, P., Prukpitikul, S., Lee, S. H., Lai, J. W., Guerin, C., Sanchez, J., Hansen, B., Grilli, S. and Matta, K. S.: The Global High Frequency Radar Network, Front. Mar. Sci., 6, 164, https://doi.org/10.3389/fmars.2019.00164, 2019.
Rotllán-García, P.: High Frequency Radar data visualization Copernicus Marine Service [code], https://doi.org/10.17882/80874, 2021.
Rubio, A., Mader, J., Corgnati, L., Mantovani, C., Griffa, A., Novellino, A., Quentin, C., Wyatt, L., Schulz-Stellenfleth, J., Horstmann, J., Lorente, P., Zambianchi, E., Hartnett, M., Fernandes, C., Zervakis, V., Gorringe, P., Melet, A., and Puillat, I.: HF radar activity in European coastal seas: next steps towards a pan-European HF radar network, Front. Mar. Sci., 20, 8, https://doi.org/10.3389/fmars.2017.00008, 2017.
Rubio, A., Reyes, E., Mantovani, C., Corgnati, L., Lorente, P., Solabarrieta, L., Mader, J., Fernandez, V., Pouliquen, S., Novellino, A., Karstensen, J., and Petihakis, G.: European High Frequency Radar network governance, EuroSea Deliverable, D3.4, EuroSea, 41 pp., https://doi.org/10.3289/eurosea_d3.4, 2021.
Ryabinin, V., Barbière, J., Haugan, P., Kullenberg, G., Smith, N., McLean, C., Troisi, A., Fischer, A., Aricò, S., Aarup, T., Pissierssens, P., Visbeck, M., Oksfeldt Enevoldsen, H., and Rigaud, J.: The UN Decade of Ocean Science for Sustainable Development, Front. Mar. Sci., 6, 470, https://doi.org/10.3389/fmars.2019.00470, 2019.
Rypina, I. I., Kirincich, A. R., Limeburner, R., and Udovydchenkov, I. A.: Eulerian and Lagrangian Correspondence of High-Frequency Radar and Surface Drifter Data: Effects of Radar Resolution and Flow Components, J. Atmos. Ocean. Tech., 31, 945–966, 2014.
Sánchez-Arcilla, A., Staneva, J., Cavaleri, L., Badger, M., Bidlot, J., Sorensen, J. T., Hansen, L. B., Martin, A, Saulter, A., Espino, M., Miglietta, M. M., Mestres, M., Bonaldo, D., Pezzutto, P., Schulz-Stellenfleth, J., Wiese, A., Larsen, X., Carniel, S., Bolaños, R., Abdalla, S., and Tiesi, A.: CMEMS-Based Coastal Analyses: Conditioning, Coupling and Limits for Applications, Front. Mar. Sci., 8, 604741, https://doi.org/10.3389/fmars.2021.604741, 2021.
Sánchez-Román, A., Ruiz, S., Pascual, A., Mourre, B., and Guinehut, S.: On the mesoscale monitoring capability of Argo floats in the Mediterranean Sea, Ocean Sci., 13, 223–234, https://doi.org/10.5194/os-13-223-2017, 2017.
Sarantis, S., Alvarez-Fanjul, E., and Coppini, G.: MONGOOS science and strategy plan, Edt: Puertos del Estado, Avenida del Partenón, 10, 28042, Madrid, Spain, ISBN 978-84-88740-08-3, 2018.
Saviano, S., Kalampokis, A., Zambianchi, E., and Uttieri, M.: A year-long assessment of wave measurements retrieved from an HF radar network in the Gulf of Naples (Tyrrhenian Sea, Western Mediterranean Sea), J. Oper. Oceanogr., 12, 1–15, https://doi.org/10.1080/1755876X.2019.1565853, 2019.
Saviano, S., De Leo, F., Besio, G., Zambianchi, E., and Uttieri, M.: HF Radar Measurements of Surface Waves in the Gulf of Naples (Southeastern Tyrrhenian Sea): Comparison with Hindcast Results at Different Scales, Front. Mar. Sci., 7, 492, https://doi.org/10.3389/fmars.2020.00492, 2020a.
Saviano, S., Cianelli, D., Zambianchi, E., Conversano, F., and Uttieri, M.: An integrated reconstruction of the multiannual wave pattern in the Gulf of Naples (South-Eastern Tyrrhenian Sea, Western Mediterranean Sea), J. Mar. Sci. Eng., 8, 372, https://doi.org/10.3390/jmse8050372, 2020b.
Saviano, S., Esposito, G., Di Lemma, R., de Ruggiero, P., Zambianchi, E., Pierini, S., Falco, P., Buonocore, B., Cianelli, D., and Uttieri, M.: Wind Direction Data from a Coastal HF Radar System in the Gulf of Naples (Central Mediterranean Sea), Remote Sens., 13, 1333, https://doi.org/10.3390/rs13071333, 2021.
Saviano, S., Biancardi, A. A., Uttieri, M., Zambianchi, E., Cusati, L.A., Pedroncini, A., Contento, G., and Cianelli, D.: Sea Storm Analysis: Evaluation of Multiannual Wave Parameters Retrieved from HF Radar and Wave Model. Remote Sens. 2022, 14, 1696, https://doi.org/10.3390/rs14071696, 2022.
Schmidt, R.: Multiple emitter location and signal parameter estimation, IEEE Trans. Antennas Propag., 3, 276–280, 1986.
Serra, M., Sathe, P., Rypina, I., Kirincich, A., Ross, S.H., Lermusiaux, P., Allen, A., Peacock, T., and Haller, G.: Search and rescue at sea aided by hidden flow structures, Nat. Commun., 11, 2525, https://doi.org/10.1038/s41467-020-16281-x, 2020.
Shen,W., Gurgel, K. W., Voulgaris, G., and Schlick, T., and Stammer, D.: Wind-speed inversion from HF radar first-order backscatter signal, Ocean. Dyn., 62, 105–121, 2012.
Shen, W. and Gurgel, K.-W.: Wind direction inversion from narrow-beam HF Radar backscatter signals in low and high wind conditions at different radar frequencies, Remote. Sens, 10, 1480, https://doi.org/10.3390/rs10091480, 2018.
Siddons, L. A., Wyatt, L. R., and Wolf, J.: Assimilation of HF radar data into the SWAN wave model, J. Mar. Syst., 77, 312–324, 2009.
Sotillo, M. G., Garcia-Ladona, E., Orfila, A., Rodríguez-Rubio, P., Maraver, J. C., Conti, D., Padorno, E., Jiménez, J. A., Capó, E., Pérez, F., Sayol, J. M., de los Santos, F. J., Amo, A., Rietz, A., Troupin, C., Tintore, J., and Álvarez-Fanjul, E.: The MEDESS-GIB database: tracking the Atlantic water inflow, Earth Syst. Sci. Data, 8, 141–149, https://doi.org/10.5194/essd-8-141-2016, 2016.
Soussi, A., Bersani, C., Sacile, R., Bouchta, D., El Amarti, A., Seghiouer, H., Nachite, D., and Al Miys, J.: Coastal Risk Modelling for Oil Spill in The Mediterranean Sea, Advances in Science, Technol. Eng. Syst. J., 5, 273–286, 2020.
Spalding, M. D., Ruffo, S., Lacambra, C., Meliane, I., Hale, L. Z., Shepard, C. C., and Beck, M. W.: The role of ecosystems in coastal protection: Adapting to climate change and coastal hazards, Ocean Coast. Manage., 90, 50–57, 2014.
Stewart, R. H. and Joy, J. W.: HF radio measurements of surface currents, Deep Sea Res.-Ocean., 21, 1039–1049, 1974.
Summers, J. K., Harwell, L. C., Smith, L. M., and Buck, K. D.: Measuring Community Resilience to Natural Hazards: The Natural Hazard Resilience Screening Index (NaHRSI)-Development and Application to the United States, Geohealth, 2, 372–394, 2018.
Teruzzi, A., Bolzon, G., Salon, S., Lazzari, P., Solidoro, C., and Cossarini, G.: Assimilation of coastal and open sea biogeochemical data to improve phytoplankton simulation in the Mediterranean Sea, Ocean Modell., 132, 46–60, https://doi.org/10.1016/j.ocemod.2018.09.007, 2018.
Tian, Z., Wen, B., Jin, L., and Tian, Y.: Radio frequency interference suppression algorithm in spatial domain for compact high-frequency radar, IEEE Geosci. Remote Sens. Lett., 15, 102–106, 2017.
Tintoré, J., Pinardi, N., Álvarez-Fanjul, E., et al.: Challenges for Sustained Observing and Forecasting Systems in the Mediterranean Sea, Front. Mar. Sci., 6, 568, https://doi.org/10.3389/fmars.2019.00568, 2019.
Trincardi, F., Cappelletto, M., Barvanti, A., Cadiou, J.-F., Bataille, A., Campillos Llanos, M., Chacón Campollo, E., and Trujillo Quintela, A.: BlueMED preliminary implementation plan D2.9 (Core Document), European Union's Horizon 2020, 2020.
Tuel, A. and Eltahir, E. A. B.: Why Is the Mediterranean a Climate Change Hot Spot?, J. Climate, 33, 5829–5843, https://doi.org/10.1175/JCLI-D-19-0910.1, 2020.
Turicchia, E., Cerrano, C., Ghetta, M., Abbiati, M., and Ponti, M.: MedSens index: The bridge between marine citizen science and coastal management, Ecol. Ind., 122, 107296, https://doi.org/10.1016/j.ecolind.2020.107296, 2021.
Updyke, T.: A study of HF Radar Outages in the Mid-Atlanti,. Galveston: Radiowave Operators Working Group (ROWG), 2017.
Vandenbulcke, L., Beckers, J. M., and Barth, A.: Correction of inertial oscillations by assimilation of HF radar data in a model of the Ligurian Sea, Ocean Dynam., 67, 117–135, https://doi.org/10.1007/s10236-016-1012-5, 2017.
Vesecky, J. F., Drake, J., Teague, C. C., Ludwig, F. L., Davidson, K., and Paduan, J.: Measurement of wind speed and direction using multifrequency HF radar, IEEE T. Geosci. Remote, 3, 1899–1901, https://doi.org/10.1109/IGARSS.2002.1026293, 2002.
Viitak, M., Maljutenko, I., Alari, V., Suursaar, U., Rikka, S., and Lagemaa, P.: The impact of surface currents and sea level on the wave field evolution during St. Jude storm in the eastern Baltic Sea, Oceanologia, 58, 176–186, 2016.
Von Schuckmann, K. et al.: Copernicus Marine Service Ocean State Report, Issue 4, J. Oper. Oceanogr., 13, 1–172, https://doi.org/10.1080/1755876X.2020.1785097, 2020.
Wang, W., Forget, P., and Guan, C.: Inversion of swell frequency from a 1-year HF radar dataset collected in Brittany (France), Ocean Dynam., 116, 1447–1456, https://doi.org/10.1007/s10236-014-0759-9, 2014.
Waters, J., Wyatt L. R., Wolf, J., and Hines, A.: Data assimilation of partitioned HF radar wave data into Wavewatch III, Ocean Modell., 72, 17–31, 2013.
Whelan, C., Teague, C., Barrick, D., Emery, B., and Washburn, L.: HF Radar Calibration with Automatic Identification System Ships of Opportunity, Phase II Final Report, NOAA SBIR, Silver Spring, MD, USA, 2013.
Wolff, C., Vafeidis, A. T., Muis, S., Lincke, D., Satta, A., Lionello, P., Jimenez, J. A., Conte, D., and Hinkel, J. A.: Mediterranean coastal database for assessing the impacts of sea-level rise and associated hazards, Sci. Data, 5, 180044, https://doi.org/10.1038/sdata.2018.44, 2018.
Wolff, C., Nikoletopoulos, T., Hinkel, J., and Vafeidis, A. T.: Future urban development exacerbates coastal exposure in the Mediterranean, Sci. Rep., 10, 14420, https://doi.org/10.1038/s41598-020-70928-9, 2020.
Wyatt, L. R.: A Relaxation Method for Integral Inversion Applied to HF Radar Measurement of the Ocean Wave Directional Spectrum, Int. J. Remote Sens., 11, 1481–1494, https://doi.org/10.1080/01431169008955106, 1990.
Wyatt, L. R.: An evaluation of wave parameters measured using a single HF radar system, Can. J. Remote Sens., 28, 205–218, https://doi.org/10.5589/m02-018, 2002.
Wyatt, L. R., Liakhovetski, G., Graber, H. C., and Haus, B. K.: Factors Affecting the Accuracy of SHOWEX HD Radar Wave Measurements, J. Atmos. Ocean. Tech., 22, 847–859, https://doi.org/10.1175/JTECH1728.1, 2005.
Wyatt, L. R., Green, J. J., Middleditch, A., Moorhead, M. D., Howarth, J., Holt M., and Keogh, S.: Operational Wave, Current, and Wind Measurements with the Pisces HF Radar, J. Ocean. Eng., 31, 819–834, 2006.
Wyatt, L. R. and Green, J. J.: Measuring High and Low Waves with HF Radar, in: Proceedings of OCEAN_EUROPE, 1–5, Bremen, Germany, 11–14 May, https://doi.org/10.1109/OCEANSE.2009.5278328, 2009.
Wyatt, L. R.: A comparison of scatterometer and HF radar wind direction measurements, J. Oper. Oceanogr., 11, 54–63, https://doi.org/10.1080/1755876X.2018.1443625, 2018.
Zambianchi, E., Uttieri, M., Cianelli, D., and Saviano, S.: HF radar data from the Gulf of Naples (Central Tyrrhenian Sea, Western Mediterranean Sea), Stazione Zoologica Anton Dohrn Napoli, [data set], https://meteo.uniparthenope.it/it/instruments/codar, last access: 21 April 2022.
Zeng, Y., Zhou, H., Roarty, H., and Wen, B.: Wind Speed Inversion in High Frequency Radar Based on Neural Network, Int. J. Antenn. Propag., 2016, 8, https://doi.org/10.1155/2016/2706521, 2016.
Zeng, Y., Zhou, H., Lai, Y., and Wen, B.: Wind-direction mapping with a modified wind spreading function by broad-beam high-frequency radar, IEEE Geosci. Remote Sens. Lett., 15, 679–683, 2018.
Zeng, Y., Zhou, H., Huang, W., and Wen, B.: Studying wave-current interaction by HF radar, IEEE conference paper: OCEANS 2019, Marseille, France, https://doi.org/10.1109/OCEANSE.2019.8867053, 17–20 June 2019.
Zervakis, V., Kokkini, Z., and Potiris, E.: Estimating Mixed Layer Depth with the use of a Coastal High-Frequency Radar, Cont. Shelf Res., 149, 4–16, https://doi.org/10.1016/j.csr.2016.07.008, 2017.
Zhou, H. and Wen, B.: Wave Height Extraction from the First-Order Bragg Peaks in High-Frequency Radars, IEEE T. Geosci. Remote, 12, 2296–2300, https://doi.org/10.1109/LGRS.2015.2472976, 2015.
- Abstract
- The Mediterranean Sea coastal regions: science priorities and societal needs
- HFR systems in MONGOOS: valuable assets for operational coastal oceanography
- HFR systems in the Mediterranean Sea
- Multi-institutional collaborative projects with HFRs in the Mediterranean Sea
- Future challenges and prospects
- Summary and conclusions
- Code availability
- Data availability
- Author contributions
- Competing interests
- Disclaimer
- Special issue statement
- Acknowledgements
- Financial support
- Review statement
- References
- Abstract
- The Mediterranean Sea coastal regions: science priorities and societal needs
- HFR systems in MONGOOS: valuable assets for operational coastal oceanography
- HFR systems in the Mediterranean Sea
- Multi-institutional collaborative projects with HFRs in the Mediterranean Sea
- Future challenges and prospects
- Summary and conclusions
- Code availability
- Data availability
- Author contributions
- Competing interests
- Disclaimer
- Special issue statement
- Acknowledgements
- Financial support
- Review statement
- References