the Creative Commons Attribution 4.0 License.
the Creative Commons Attribution 4.0 License.
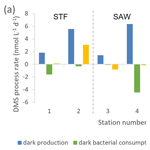
Dimethyl sulfide cycling in the sea surface microlayer in the southwestern Pacific – Part 2: Processes and rates
Alexia D. Saint-Macary
Andrew Marriner
Stacy Deppeler
Karl A. Safi
As the sea surface microlayer (SML) is the uppermost oceanic layer and differs in biogeochemical composition to the underlying subsurface water (SSW), it is important to determine whether processes in the SML modulate gas exchange, particularly for climate active gases. Enrichment of dimethyl sulfide (DMS) and its precursor dimethylsulfoniopropionate (DMSP) has been reported in the SML, but it remains unclear how this is maintained whilst DMS is lost to the atmosphere. To examine this, a comprehensive study of DMS source and sink processes, including production, consumption, and net response to irradiance, was carried out in deck-board incubations of SML water at five locations in different water masses in the southwestern Pacific east of New Zealand. Net consumption of DMSP and production of DMS in the light and dark occurred at all sites. The net response of DMS and DMSP to irradiance varied between stations but was always lower than conversion of DMSP to DMS in the dark. In addition, DMS photolytic turnover was slower than reported elsewhere, which was unexpected given the high light exposure in the SML incubations. Although no relationships were apparent between DMS process rates and biogeochemical variables, including chlorophyll a, bacteria, and phytoplankton groups, net bacterial DMSP consumption was correlated with DMSP and DMS concentrations and also dinoflagellate and Gymnodinium spp. biomass, supporting the findings of a companion study that dinoflagellates play an important role in DMS cycling in the SML. However, net DMS production rates and accumulation were low relative to regional air–sea DMS loss, indicating that DMS cycling within the SML is unlikely to influence regional DMS emissions.
- Article
(1870 KB) - Full-text XML
-
Supplement
(440 KB) - BibTeX
- EndNote
The climate-active trace gas dimethyl sulfide (DMS) is the primary natural sulfate aerosol precursor (Yu and Luo, 2010; Leaitch et al., 2013; Park et al., 2017; Sanchez et al., 2018) that has been hypothesized to contribute to the regulation of climate via formation of cloud condensation nuclei (Charlson et al., 1987; Quinn and Bates, 2011). DMS concentration in the surface mixed layer and emission to the atmosphere is the net result of production and consumption by various biological, photochemical, and physical processes (Stefels et al., 2007). DMS is mainly produced by enzymatic cleavage of its precursor dimethylsulfoniopropionate (DMSP), with acrylate as the other product (Keller et al., 1989; Stefels et al., 2007). DMSP concentration in seawater is determined by phytoplankton biomass and speciation (Keller et al., 1989) and also bacterial composition and production (Curson et al., 2017) and occurs in dissolved and particulate forms, with the latter accounting for ∼ 80 % of total DMSP (Keller and Korjeff-Bellows, 1996; Belviso et al., 2000; Yang et al., 2005a; Zhang et al., 2009). It is the dissolved DMSP that constitutes the source for bacterial conversion to DMS, but phytoplankton also release DMS directly during senescence-related cell-lysis (Yoch, 2002; Stefels, 2000). There are at least four independent pathways by which DMSP can be degraded enzymatically by bacteria, three of which lead to the production of DMS; yet, DMS production represents only 5 % to 10 % of the available DMSP, as the primary DMSP removal pathway of bacterial demethylation results in production of methanethiol (MeSH) (Kiene and Linn, 2000). As DMS production is largely influenced by phytoplankton type and density, its concentration in the euphotic zone generally reflects the vertical distribution of primary production and biomass, with a DMS maximum in near-surface waters and concentration decreasing with depth (Dacey et al., 1998; Bouillon et al., 2002; Rellinger et al., 2009). The main DMS sink in the surface mixed layer is biological consumption, which accounts for 50 % to 88 % (Galí et al., 2013), with photochemical oxidation and emission to the atmosphere accounting for 8 %–34 % and 4 %–16 % of DMS loss, respectively (Galí and Simó, 2015). Both production and loss processes are in turn influenced by environmental drivers, such as irradiance, nutrient concentration, temperature, and pH, resulting in regional and temporal variation in DMS concentration (Stefels et al., 2007).
The sea surface microlayer (SML) plays a key role in air–sea gas exchange as the interface between the ocean and the atmosphere. It is a very thin layer (1–1000 µm) with differing physicochemical and biological properties to the underlying water (Hunter, 1980), including elevated concentrations of carbohydrates, proteins, and lipids (Sieburth, 1983; Cunliffe et al., 2013). The SML is more biologically active than the underlying subsurface water (SSW), due to high bacterial activity and abundance (Cunliffe et al., 2011). Elevated respiration by bacterioneuston in the SML is reflected in O2 and CO2 emissions (Reinthaler et al., 2008) and altered cycling of trace gases such as CH4, H2, N2O, and CO (Sieburth, 1983; Conrad and Seiler, 1988; Upstill-Goddard et al., 2003). The SML also contributes to possible climate regulation when emitted trace gases are oxidized, leading to secondary organic aerosol, which may influence atmospheric radiative properties (Leck and Bigg, 2005; Roslan et al., 2010). Dissolved DMSP is often enriched in the SML (Yang et al., 2005b; Yang and Tsunogai, 2005; Yang et al., 2005a; Zhang et al., 2008; Matrai et al., 2008; Zhang et al., 2009; Yang et al., 2009), potentially due to stabilization by dissolved organic substances (Gibbs adsorption surface, Adamson and Gast, 1967) and high surface tension, which energetically favours dissolved DMSP adsorption to dissolved organic substances (Zhang et al., 2008, 2009). As a result of elevated dissolved DMSP and enrichment of bacterioneuston in the SML (Sieburth, 1983), DMS production from enzymatic cleavage and consumption is also elevated in the SML relative to SSW (Yang et al., 2001, 2005a, b; Yang and Tsunogai, 2005; Zhang et al., 2008). DMS enrichment in the SML is often associated with underlying phytoplankton blooms dominated by DMSP-producers (Walker et al., 2016) and with high phytoplankton biomass in general (> 2 mg m−3, Yang et al., 2005a; Zhang et al., 2009). Indeed, DMS enrichment in the SML may require specific biological, biogeochemical, and meteorological conditions, which may result in anomalously high air–sea DMS flux in regions of high productivity (Walker et al., 2016). However, understanding of the factors that maintain DMS enrichment in the SML is limited, particularly as few studies have examined the biogeochemical composition of the SML.
Drivers of DMSP and DMS cycling are more intense in the SML than the SSW. Wind increases ventilation of DMS from the SML (Yang et al., 2001; Yang and Tsunogai, 2005; Yang et al., 2005a; Zhang et al., 2008) and may also concentrate particulate organic material in surface patches that act as hotspots for DMS cycling. In addition, incident light and ultraviolet (UV) exposure are greater in the absence of water column attenuation (Hardy, 1982; Stolle et al., 2020), which may, directly and indirectly, influence DMSP and DMS (Zemmelink et al., 2005, 2006). DMS photo-oxidation to dimethyl sulfoxide (DMSO) under a full light spectrum (Kieber et al., 1996) is enhanced in the presence of photosensitizers, such as chromophoric dissolved organic matter (CDOM) (Brimblecombe and Shooter, 1986; Brugger et al., 1998; Vogt and Liss, 2009), which is generally enriched in the SML (Frew et al., 2002, 2004). Conversely, this may also limit light exposure as organic matter enrichment and gel particles may attenuate UV and photosynthetically active radiation (PAR) in the SML (Bailey et al., 1983; Carlucci et al., 1985; Agogué et al., 2005). Irradiance represents a SML sink for DMS via photo-oxidation but also stimulates intracellular production of DMSP in phytoplankton under light stress and inhibits the bacterial consumption of DMS (Sunda et al., 2002). Consequently, light may enhance both DMS production and consumption, and thus the net effect of these processes may be particularly significant in the SML. Although solar radiation dose is an important factor in determining temporal and spatial variability of DMS in surface water (Simó and Pedrós-Alió, 1999; Simó and Dachs, 2002; Vallina and Simó, 2007; Miles et al., 2009), only two previous studies have considered the impact of light on DMSP and DMS in the SML (Zemmelink et al., 2005, 2006).
In situ measurements in the SML and SSW in southwestern Pacific waters during the Sea2Cloud voyage identified only minor DMSP and DMS enrichment (see Saint-Macary et al., 2022, hereafter referred to as S-M1), in contrast to a previous southwestern Pacific regional study (Walker et al., 2016) and also measurements in other regions, as synthesized in Walker et al. (2016). The apparent absence of DMS enrichment in the same region, as determined by a new technique for sampling DMS in the SML (S-M1), and the requirement for high DMS production to maintain SML enrichment relative to ventilation losses (Walker et al., 2016) highlights the need for process studies of DMS in the SML. In this paper, SML process rates were measured in deck-board incubations of SML water from five stations across different water masses in the southwestern Pacific east of New Zealand to determine the controls of DMSP and DMS and ultimately the significance of DMS cycling in the SML.
2.1 Regional setting
The Sea2Cloud voyage took place from the 16 to 28 March 2020 (austral autumn) around the Chatham Rise, east of New Zealand, onboard R/V Tangaroa (Fig. 1). The characteristics of the water masses sampled during this voyage and meteorological conditions are summarized in Table 1. Six workboat deployments were carried out to sample the SML and SSW in different water mass types: subtropical front (STF) at stations 1 and 2, subantarctic water (SAW) at stations 3 and 4, the subtropical water (STW) at station 5, and mixed water (Mixed) at station 6 (see Fig. 1, Table 1). The mixed water had elevated nutrient content relative to the STW, reflecting a mixture of coastal and shelf water with STW. Station 5-STW was sampled for SML characterization (S-M1) but had no deck-board incubation as sampling occurred in the afternoon (in contrast to the other stations). A sipper consisting of a silicon tube with multiple inlets (internal diameter 2.2 mm) that floated on the surface of the water enabled sampling of 2.4 L of water from the SML by peristaltic pump or syringe from a silicon tube (internal diameter 2.2 mm) (see S-M1).
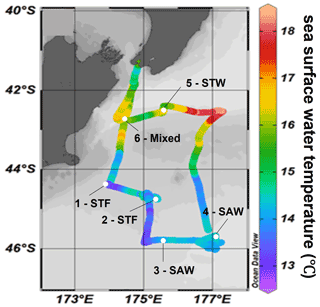
Figure 1Sea2Cloud voyage track with workboat station positions overlain on sea surface water temperature (∘C). Figure plotted using Ocean Data View (Schlitzer, 2020). The grey shading shows the undersea topography, with the darker grey band along 43.5∘ S indicating the Chatham Rise. Station 5-STW was sampled for SML characterization (S-M1) but had no deck-board incubation.
2.2 Deck incubation set-up
The SML water was transferred by gravity into pre-rinsed and flushed 6 × 250 mL UV transparent borosilicate glass bulbs that transmit 90 % of UV-A and UV-B, with the bulbs filled completely to eliminate any headspace. The bulbs were incubated in a shallow 37 L seawater bath (17 cm depth), half-immersed in continually flowing surface water to maintain temperature whilst maximizing irradiance to mimic the SML. A PAR light Odyssey® photosynthetic irradiance recording system was placed next to the deck incubation to measure incoming irradiance in the wavelength range 400–700 nm. However, the variation in PAR between experiments was not considered in the interpretation.
Each of the five deck incubations was of 6 h duration (from 12:00 or 14:00 to 18:00 or 20:00 NZDT) and used DMS process rate measurement techniques (Simó et al., 2000; Yang et al., 2005b; Yang and Tsunogai, 2005; Yang et al., 2001) modified for small water volumes. Three treatments were each carried out in duplicate. The first pair of bulbs, in set A, were exposed to ambient deck irradiance to simulate in situ conditions in the SML but excluded air–sea loss. The second treatment, in set B, was maintained in the dark with the bulbs covered by black tape. Exclusion of light eliminated DMS photo-oxidation and light stress and provided an estimate of the net biological effect on DMSP and DMS in the dark. Light was also excluded in set C, which included the addition of DMS-free dimethyl disulfide (DMDS), an inhibitor of DMS bacterial consumption, at a final concentration of 200 nmol L−1 (Wolfe and Kiene, 1993), so providing a dark DMS production rate.
2.3 DMSP and DMS analytical system
Time zero (T0) DMSP and DMS concentrations were determined from the original water sample. After 6 h incubation, water was sub-sampled from the borosilicate bulbs into 118 mL amber bottles for DMSP and DMS analysis. For DMS measurements, water from the amber bottles was withdrawn in plastic Terumo® syringes and injected through a 25 mm glass microfiber filter (GF/F) into a 1 mL loop, before transfer to a silanized sparging tower where the sample was sparged for 5 min with nitrogen (N2) at a flow rate of 50 mL min−1. Nafion® dryers removed water vapour from the gas samples before DMS pre-concentration at −110 ∘C on a Tenax® TA trap. The trap was then heated to 120 ∘C to release the DMS into an Agilent Technology 6850 gas chromatograph (DB-megabore sulfur chemiluminescent detector column, 70 m length, 0.530 megabore diameter, and film thickness 4.30 µm) coupled to an Agilent 355 sulfur chemiluminescent detector (GC-SCD). The detector's daily sensitivity and detection limit were confirmed using VICI® methyl ethyl sulfide and DMS permeation tubes, with an average detection limit of 0.14 (± 0.03) pgS s−1. For DMSP measurements, 20 mL glass vials were filled and two pellets of NaOH added to hydrolyse DMSP to DMS before gas-tight sealing the vials. DMSP samples were stored in the dark at ambient temperature with analysis of the liberated DMS within 24 h of sampling, using the semi-automated purge and trap system and GC-SCD as described above. A wet standard calibration curve was made daily from a stock solution of DMSP diluted in Milli-Q® water, with DMS calibration concentrations ranging from 0.1 to 95 nmol L−1. These were decanted into 20 mL gas-tight glass vials, hydrolysed with two pellets of NaOH, and then injected into the sparging unit and processed as with the samples.
2.4 Rate calculation
The rate of change in DMSP and DMS concentration, k, was calculated from the linear slope between T0 and T6 and converted to per day rates (nmol L−1 d−1), with turnover time (day) subsequently calculated by dividing the initial DMS or DMSP concentration by the rate, as described in Table 2. The incubation design had some limitations, with only two data points and a short incubation time; however, the 6 h period was compatible with natural light availability and minimized bottle effects. The net irradiance response of DMSP, kDMSP ir, and DMS, kDMS ir, were calculated as the differences between the set exposed to light (A) and dark (B). The DMSP dark bacterial consumption rate, kDMSP cn, was calculated using the change in DMSP concentration in set B over the 6 h incubation. DMSP dark bacterial consumption rate has been previously calculated using a first-order loss rate constant as the slope of the natural log of DMSP concentration versus time (Kiene, 1996); however, as there were only two time points (T0 and T6), the slope of the linear decrease in DMSP concentration was used in the current study. The DMS dark bacterial consumption rate, kDMS cn, was calculated as the difference between the dark sets (C) and (B), i.e. with and without DMDS addition, respectively (Yang et al., 2001, 2005b). The DMS dark production rate, kDMS pr, was estimated as the change in DMS concentration in the DMDS-treated samples in set (C) (Yang et al., 2001, 2005b; Simó et al., 2000). DMS dark yield was calculated as the ratio between the DMS dark production rate and the DMSP dark bacterial consumption rate. Process rates were compared with the calculated DMS air–sea flux (S-M1), and a DMS air–sea turnover, , was also generated by relation to the initial DMS concentration in the SML.
Table 2Definition and calculation of DMSP and DMS process rates in nmol L−1 d−1 and turnover in days (d) and minutes for air–sea turnover.
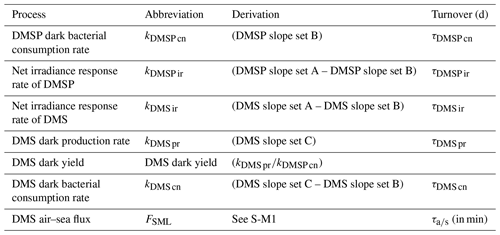
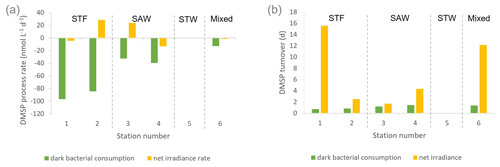
Figure 2(a) DMSP process rates (nmol L−1 d−1) and (b) DMSP consumption and net irradiance turnover (d) determined in deck incubation of SML water at each station. Water mass type is indicated by the label at the top of the figure and is also separated by vertical dashed lines. Station 5-STW was sampled for SML characterization (S-M1) but had no deck-board incubation.
2.5 Statistical analysis
The Shapiro test was used to verify the normality of variable distribution (see results in Supplement Table S1). For the non-normally distributed variables Spearman's rank correlation was carried out, and for the normally distributed data a Pearson test was applied. Linear correlation was considered significant where the coefficient of correlation (rho for Spearman's rank and r for Pearson test) was higher than 0.5 and the p value was lower than 0.05.
3.1 DMSP process rates
DMSP concentrations decreased over the 6 h incubation in all treatments, with the highest losses at the frontal stations 1 and 2 and lowest at 3-SAW and 6-Mixed (Supplement Fig. S1). The DMSP loss was generally similar for all treatments within each station, except for 3-SAW (which showed higher DMSP loss in set B). Although variable between stations, kDMSP ir was negative at three stations (range: −13 to +29 nmol L−1 d−1; average: +7 nmol L−1 d−1), with the lowest rate at 4-SAW and highest at 2-STF (Fig. 2a). The DMSP dark bacterial consumption rate was generally greater than kDMSP ir, with an average loss of 53 nmol L−1 d−1 (range: 13–97 nmol L−1 d−1), with the lowest rates at 6-Mixed and highest in STF waters. As a result, there was a net loss of DMSP at all stations (average 47 nmol L−1 d−1; range: 9–101 nmol L−1 d−1). The DMSP data from the deck incubation are summarized in Table 3, with the rates also considered in terms of turnover of DMSP concentration in the SML (T0 concentration), as described in Sect. 2. DMSP dark bacterial consumption turnover (τDMSP cn) was faster than τDMSP ir with average values of 1.1 d (range: 0.7–1.4 d) and 7.3 d (1.7–16 d), respectively (Fig. 2b). τDMSP cn was fastest in STF water but relatively uniform across the other stations, whereas τDMSP ir did not show any pattern in relation to water mass. Overall, only kDMSP cn was correlated to biogeochemical variables in the SML, including dinoflagellate and Gymnodinium biomasses, DMSP and DMS concentrations, and the > 50 µm phytoplankton size fraction (Supplement Table S1).
3.2 DMS process rates
In contrast to DMSP, DMS concentration increased in all incubations with significant differences between stations (Supplement Fig. S2). Station 2-STF showed the largest increase in DMS in set A relative to T0 (8 nmol L−1 d−1), whereas there were only minor increases at 1-STF and 6-Mixed (< 1 nmol L−1 d−1). There were also variations within stations, with DMS increases in the dark treatment (set B) at 2-STF, 3-SAW, and 4-SAW (Supplement Fig. S2). Dark production was the dominant DMS process at an average of 3 nmol L−1 d−1, exceeding kDMS cn and kDMS ir at all stations except 6-Mixed (Fig. 3a). The kDMS cn varied from 0 to 4.44 nmol L−1 d−1 and was higher at 1-STF and 4-SAW. kDMS ir was positive and also highest at 2-STF, whereas it was negative at 3-SAW and 4-SAW. DMS dark yield was on average 6 %, with a maximum yield at 4-SAW (16 %) and a minimum at 6-Mixed (1.4 %, Table 4).
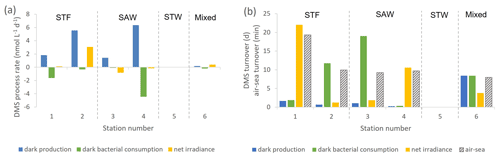
Figure 3(a) DMS process rates (nmol L−1 d−1) at each sampling station. (b) DMS turnover time in days for dark production, dark bacterial consumption, and net irradiance response and in minutes for air–sea turnover, with water mass type indicated by the label at the top of the figure and separated by vertical dashed lines. Station 5-STW was sampled for SML characterization (S-M1) but had no deck-board incubation.
The DMS rates were assessed in relation to DMS concentration to generate a turnover, as described in Sect. 2 and summarized in Table 4. τDMS cn varied between 0.4 and 19 d, with an average of 8.3 d (Fig. 3b), and was generally similar to τDMS ir (average 7.9 d; range 1.2–22 d). DMS dark production turnover was faster than τDMS ir and τDMS cn at all stations, except 6-Mixed, at an average of 3 d (range: 0.3–8.4 d). However, the air–sea turnover τair-sea, calculated from the air–sea flux (S-M1), was considerably shorter at an average of 11 min (8–19 min), and so it was ∼ 1100-fold faster than τDMS pr. In addition, DMS process rates and turnover did not show any significant correlations with ancillary variables (Chl a, phytoplankton community composition, and bacterial abundance (S-M1) in Supplement Table S1).
Table 3Summary of DMSP process rates (nmol L−1 d−1) and turnover (d) in SML water for each station. The DMSP concentration is in nmol L− 1. The calculation details for the rates and turnovers are given in Table 2. Results are the mean value of duplicate incubations.
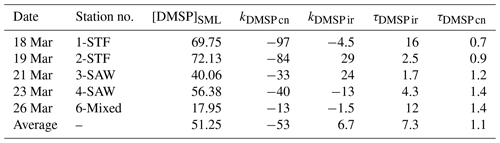
3.3 DMS : DMSP ratio
The DMS : DMSP ratio in sets A (light) and B (dark) were compared to the initial in situ DMS : DMSP in the SML (Fig. 4). DMS : DMSP was on average 0.05 in the SML (range: 0.03–0.08) and increased to 0.07 in the absence of air–sea loss in the incubations in both sets A and B (ranges 0.04–0.09 and 0.05–0.08, respectively). DMS : DMSP was similar in sets A and B at each station, except for 3-SAW where it was higher in set B (dark).
The characteristics of the SML during the current study contrasted with a previous southwestern Pacific regional study (Walker et al., 2016) and results from other regions (Nguyen et al., 1978; Yang, 1999; Yang and Tsunogai, 2005; Yang et al., 2005a; Zhang et al., 2009; Zemmelink et al., 2006), with only limited enrichment of DMSP, DMS, and Chl a at one of six stations (S-M1). Consequently, the DMS air–sea flux was not significantly affected by DMS in the SML (S-M1) and was generally consistent with the climatological estimates of Lana et al. (2011) and Wang et al. (2020). Although DMS dark production was the dominant process in the deck-board incubations, net DMS accumulation was low (Table 4), which combined with the reported variation in enrichment raises questions as to how excess DMS is maintained in the SML when air–sea loss is significant (Fig. 3b). The following discussion considers the processes and factors influencing DMSP and DMS cycling in the SML and whether these are sufficient to generate DMS enrichment (S-M1).
Table 5Summary of DMS and DMSP processes in the SML and potential factors influencing enrichment factor (EF) DMS in different water masses. In the EF columns, depletion is indicated by “−” and enrichment by “+”, while “n/s” stands for not significant. DMSP enrichment was measured at station 5-STW (S-M1), and thus it is not presented here. For the dominant phytoplankton group in the SML, “F” stands for dinoflagellate. For the processes, a “−” indicates DMS or DMSP loss and a “+” DMS or DMSP production, with triplicate symbols indicating the dominant DMS or DMSP transformation process at the respective station (air–sea flux was always a loss process for DMS and always exceeded other process rates). The maximum air–sea flux (> 5 µmol m−2 d−1) is indicated by two “−” signs. Results from SM-1 are indicated by *, and n/d stands for “not determined”.

4.1 DMSP processes
The current study is, to our knowledge, the first to determine DMSP process rates in the SML. DMSP loss occurred in all treatments at all stations, with the highest kDMSP cn in the diatom bloom at 2-STF. As Chl a and bacterial abundance were elevated at this station (S-M1), DMSP loss was enhanced in association with elevated biological activity; however, bacterial community composition is considered a more significant determinant of kDMSP cn than bacterial abundance (Vila-Costa et al., 2008). In the current study, kDMSP cn was correlated with SML dinoflagellate and Gymnodinium biomass and with DMS and DMSP concentrations in the SML, suggesting the importance of dinoflagellate in DMS and DMSP dynamics in the SML (Supplement Table S1, S-M1). The kDMSP cn, range of 13–97 nmol L−1 d−1 measured in the SML (Table 3) is higher than regional rates determined in SSW with the 35S-DMSP method (3–60 nmol L−1 d−1; Lizotte et al., 2017), consistent with the SML being more biologically active than the SSW (Cunliffe et al., 2011). However, this difference in regional consumption rates may reflect methodological differences, as the net concentration change method used in the current study generally delivers higher consumption rates than the dissolved 35S-DMSP method (Vila-Costa et al., 2008). That dark bacterial consumption was the dominant DMSP process is consistent with bacterial demethylation being the primary DMSP removal process in the surface ocean (Kiene and Linn, 2000).
The net response of DMSP to irradiance was variable (Fig. 2a and Table 5), as reported in other studies (Slezak et al., 2001, 2007). Exposure to light can affect intracellular synthesis of DMSP (Stefels, 2000; Hefu and Kirst, 1997), and phytoplankton DMSP production is enhanced during antioxidant response to light stress (Sunda et al., 2002). Exposure to UV and UV + PAR may have differential effects on intracellular accumulation of DMSP in the coccolithophore Emiliania huxleyi (Sunda et al., 2002; Van Rijssel and Buma, 2002; Archer et al., 2010), with DMSP synthesis inhibited under high UV radiation (Archer et al., 2018; Herndl et al., 1993; Muller-Niklas et al., 1995; Slezak et al., 2001; Sunda et al., 2002; Van Rijssel and Buma, 2002). However, UV radiation may also inhibit bacterial DMSP removal (Slezak et al., 2001) and result in DMSP accumulation. As the response of phytoplankton DMSP synthesis and bacterial cycling varies with light intensity and light exposure also varied between deck incubations, this limits interpretation of the irradiance-related processes and factors influencing DMSP cycling in the SML. Regardless, the net effect of irradiance on DMSP was minor relative to dark bacterial consumption, indicating potential for DMS production in the SML.
4.2 DMS production and bacterial consumption
DMS dark production was the dominant DMS process in the SML, whereas the net response to irradiance and dark bacterial consumption of DMS varied between stations, with no single factor responsible for this variation (Fig. 3, Table 4). DMS is produced by enzymatic cleavage of DMSP and also direct phytoplankton release (Yoch, 2002), as supported by the correlations between DMSP and DMS concentration in both the SSW and SML (S-M1). The mean τDMS pr and τDMS cn in the SML were 3 and 8.3 d, respectively, within the range reported elsewhere for the SML (0.1 to 4.2 d; Yang et al., 2005b; Yang and Tsunogai, 2005; Yang et al., 2005a; Zhang et al., 2008). In terms of DMS consumption, τDMS cn in the SML was more rapid than τDMS cn reported for subsurface water during the Surface Ocean Aerosol Production (SOAP) voyage in the same region of the southwestern Pacific (2.3 to 36.5 d); (Lizotte et al., 2017), again reflecting faster biological turnover in the SML (Yang and Tsunogai, 2005; Yang et al., 2005a; Zhang et al., 2008).
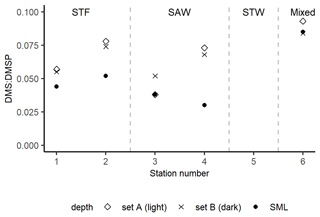
Figure 4DMS : DMSP ratio in the SML at T0 and at the end of the 6 h deck incubations in sets A (light) and B (dark) at each station. At 3-SAW, the ratio in set A is equal to (and thus obscured by) the initial ratio in the SML. Set C results are not included as bacterial consumption was inhibited by the addition of DMDS, meaning that the DMSP : DMS would not be comparable to the unperturbed set A and B. Station 5-STW was sampled for SML characterization (S-M1) but had no deck-board incubation.
4.3 Effect of irradiance on DMS
As with DMSP, the response to irradiance was variable, with a net decrease in DMS at 3-SAW and 4-SAW in set A (Fig. 3, Table 5), suggesting photo-oxidation of DMS (Brimblecombe and Shooter, 1986), and a positive effect at the other stations indicating stimulation of DMS production. This is consistent with the higher DMS : DMSP ratio in the light incubation (set A) at most stations, indicating elevated DMS production or inhibition of DMS bacterial consumption by light (Fig. 4). That the net response of DMS to irradiance was negative only at the SAW stations suggests differing sensitivity to light between water masses, although no significant relationships were identified between PAR and DMS concentration, process rates, or enrichment in the SML (S-M1, Supplement Table S1). Under light stress, phytoplankton may elevate DMS production via three pathways – overflow, antioxidant systems, and cell damage (Gali et al., 2013). Under stress, such as nitrogen-limited conditions with the overflow hypothesis (Stefels, 2000) and iron limitation with the antioxidant pathway (Sunda et al., 2002), excess intracellular DMSP is produced and released, increasing the substrate for conversion to DMS. In surface water exposed to high solar radiation with low UV attenuation, the cell damage pathway may result in increased cell permeability, further increasing DMSP availability for conversion to DMS (Gali et al., 2013). An additional impact of irradiance is the inhibition of bacterial DMS consumption (Slezak et al., 2007; Toole et al., 2006a), which may enhance DMS accumulation (Gali et al., 2013). As the net response of DMS to irradiance was more often positive (Fig. 3, Table 5), this indicates that biological responses, such as stress production of DMS and inhibition of DMS consumption, were greater than losses to photo-oxidation.
With the exclusion of air–sea exchange in the deck-board incubation, DMS : DMSP in sets A and B would be expected to exceed the in situ ratio in the SML, as observed at most stations (Fig. 4). Only 3-SAW showed a different trend, with a similar DMS : DMSP in set A to the in situ ratio but higher ratio in set B, potentially indicating suppression of DMS production by irradiance at this station. Determination of the DMS photolysis constant, which is the inverse of τDMS ir, generated rates of 0.004–0.035 h−1, which are significantly lower than rates reported for subsurface water (0.026–0.14 h−1 (Toole et al., 2006b; Brimblecombe and Shooter, 1986; Brugger et al., 1998; Kieber et al., 1996). This slower photolytic DMS turnover was unexpected due to the elevated solar and UV radiation exposure in the SML, although this may reflect variability of irradiance both during and between the deck incubation, in contrast to laboratory studies that use constant radiation often with wavelength cut-offs (Brimblecombe and Shooter, 1986; Brugger et al., 1998; Kieber et al., 1996; Toole et al., 2006a). In addition, previous photolysis studies have used filtered seawater (Kieber et al., 1996; Toole et al., 2006a; Brimblecombe and Shooter, 1986; Brugger et al., 1998), in contrast to the unfiltered samples in this study in which particle scattering and absorption may have buffered photolytic DMS losses. The slower photolytic DMS turnover in the current study could also be due to the SML biogeochemical properties; for example, the SML is often enriched in gel-like particles that can protect the SML compounds from high solar irradiance (Ortega-Retuerta et al., 2009).
4.4 DMS dark yield
Notwithstanding differences between the 35S-DMSP and dark net loss methodologies (Vila-Costa et al., 2008), DMS dark yields in the SML were in agreement with previous regional estimates (Lizotte et al., 2017; Vila-Costa et al., 2008). DMS dark yield was highest at 4-SAW due to high kDMS pr (Table 5), potentially due to the elevated dinoflagellate and small flagellate biomass at this station (S-M1), although no relationships were identified between DMS dark yield and other variables (see Supplement Table S1). The DMS : DMSP ratio indicated that 5 % to 10 % of DMSP was converted to DMS, consistent with previously reported estimates, supporting the hypothesis that the proportion of DMSP cleaved to DMS is relatively constant across the ocean and independent of regional influences and phytoplankton composition (Lizotte et al. (2017) and references therein). Although this is surprising considering the reported enrichment of bacteria and dissolved DMSP in the SML (Yang et al., 2009, 2005b; Zhang et al., 2008; Matrai et al., 2008), it is consistent with the general absence of enrichment in the current study (S-M1, see Table 5).
4.5 Relating SML processes to DMS enrichment
Air–sea emission was the dominant process controlling DMS concentration in the SML, with the air–sea turnover rate in the SML ranging from 8 to 19 min, which is within the range reported in other studies (0.1–24.4 min, Yang et al., 2005a; Yang and Tsunogai, 2005; Yang et al., 2001). Consequently, despite net DMS accumulation in the SML (Table 4), the significantly greater air–sea loss should deplete DMS and so eliminate SML enrichment (Table 5). As the SML DMS production rates in the current study are consistent with others reported (Yang et al., 2005b; Yang and Tsunogai, 2005; Yang et al., 2001; Zhang et al., 2008), DMS production in the SML is unlikely to match air–sea loss, and consequently regional DMS air–sea flux should not be influenced by DMS cycling in the SML (Yang and Tsunogai, 2005). Furthermore, at the single station where DMS enrichment was significant (3-SAW, S-M1), DMS production did not dominate and DMS dark yield was low (Table 5). SML DMS might interact with the organic material in the SML, which could lower DMS volatility leading to enrichment. However, there were no correlations between DMS and organic matter in the SML, and so the enrichment cannot be explained. It should be kept in mind that the apparent disconnect between SML process rates and enrichment may reflect comparison of in situ conditions with artificial conditions in the deck-board incubation. It is challenging to simulate the SML in vitro, particularly in recreating the SML thickness and interaction with the overlaying atmosphere and subsurface water, and the incubation design may have introduced artefacts (particle concentration, wall effects) and altered light exposure and attenuation relative to in situ conditions.
The current study was motivated by previous regional observations of high DMS enrichment and inferred influence of the SML on air–sea DMS emissions during the SOAP voyage (Walker et al., 2016) but has found no evidence to support this. The previous study noted the large inconsistency between estimated SML DMS production rates and the inferred production rates required to support air–sea flux estimates. This inconsistency is further confirmed by the similarity in the net DMS accumulation rate of 0.3–8 nmol L d−1 (n=5) between the current study and during SOAP (range: −1 to 11 nmol L d−1; Lizotte et al., 2017). The significant correlations in the current study (see Supplement Table S1) between kDMSP cn and DMS (; p=0.05), and also between kDMSP cn and DMSP concentration (; p=0.03), dinoflagellate biomass (; p=0.01), and Gymnodinium biomass (; p=0.05), indicate that phytoplankton community composition is an important determinant of DMSP and DMS cycling in the SML. This then supports the contention that an optimal combination of biogeochemical, physical, and meteorological factors – low winds, near-surface stratification, and a bloom of high-DMSP dinoflagellates – resulted in the significant DMS enrichment in the SML during the SOAP voyage (Walker et al., 2016), whereas these conditions were not experienced during the current study (SM-1).
The current study presents the results of a comprehensive investigation into DMSP and DMS processes in the SML that is, to our knowledge, the first to assess DMSP cycling and the net effect of irradiance on both DMSP and DMS in the SML. Bacterial consumption of DMSP and dark production of DMS were the dominant processes in the SML, with irradiance having a relatively minor impact on both species. Although these results are only representative of one region of the southwestern Pacific during the austral autumn, the combination of in situ SML observations in S-M1 and process rates in the current study indicate that DMS enrichment in the SML is rare and that net accumulation of DMS in the SML is insufficient to balance DMS air–sea loss.
For data availability please contact cliff.law@niwa.co.nz.
The supplement related to this article is available online at: https://doi.org/10.5194/os-18-1559-2022-supplement.
ADSM developed the experiment set-up with advice from CSL. ADSM wrote the manuscript and analysed DMSP and DMS. AM contributed to DMSP and DMS analysis. SD analysed samples on the Flowcam and processed results, and KS identified the species by optical microscopy. ADSM interpreted the results with guidance from CSL.
The contact author has declared that none of the authors has any competing interests.
Publisher's note: Copernicus Publications remains neutral with regard to jurisdictional claims in published maps and institutional affiliations.
This article is part of the special issue “Sea2Cloud (ACP/OS inter-journal SI)”. It is not associated with a conference.
We would like to thank Theresa Barthelmeß for her contribution to the SML sampling and Antonia Cristi and Wayne Dillon for their help during the Sea2Cloud campaign. We would also like to thank the officers and crew of the R/V Tangaroa for their support and expertise.
This research has been supported by NIWA SSIF funding to the Ocean–Climate Interactions Programme. Alexia D. Saint-Macary's PhD was funded by the University of Otago Departmental Award.
This paper was edited by Mario Hoppema and reviewed by two anonymous referees.
Adamson, A. W. and Gast, A. P.: Physical chemistry of surfaces, Interscience publishers, New York, A Wiley-Interscience, ISBN 0-471-14873-3, 1967.
Agogué, H., Casamayor, E. O., Bourrain, M., Obernosterer, I., Joux, F., Herndl, G. J., and Lebaron, P.: A survey on bacteria inhabiting the sea surface microlayer of coastal ecosystems, FEMS Microbiol. Ecol., 54, 269–280, https://doi.org/10.1016/j.femsec.2005.04.002, 2005.
Archer, S. D., Ragni, M., Webster, R., Airs, R. L., and Geider, R. J.: Dimethyl sulfoniopropionate and dimethyl sulfide production in response to photoinhibition in Emiliania huxleyi, Limnol. Oceanogr., 55, 1579–1589, https://doi.org/10.4319/lo.2010.55.4.1579, 2010.
Archer, S. D., Stefels, J., Airs, R. L., Lawson, T., Smyth, T. J., Rees, A. P., and Geider, R. J.: Limitation of dimethylsulfoniopropionate synthesis at high irradiance in natural phytoplankton communities of the Tropical Atlantic, Limnol. Oceanogr., 63, 227–242, https://doi.org/10.1002/lno.10625, 2018.
Bailey, C. A., Neihof, R. A., and Tabor, P. S.: Inhibitory effect of solar radiation on amino acid uptake in Chesapeake Bay bacteria, Appl. Environ. Microbiol., 46, 44–49, https://doi.org/10.1128/aem.46.1.44-49.1983, 1983.
Belviso, S., Christaki, U., Vidussi, F., Marty, J.-C., Vila, M., and Delgado, M.: Diel variations of the DMSP-to-chlorophyll a ratio in Northwestern Mediterranean surface waters, J. Mar. Syst., 25, 119–128, https://doi.org/10.1016/S0924-7963(00)00011-7, 2000.
Bouillon, R.-C., Lee, P. A., de Mora, S. J., Levasseur, M., and Lovejoy, C.: Vernal distribution of dimethylsulphide, dimethylsulphoniopropionate, and dimethylsulphoxide in the North Water in 1998, Deep-Sea Res. Pt. II, 49, 5171–5189, https://doi.org/10.1016/S0967-0645(02)00184-4, 2002.
Brimblecombe, P. and Shooter, D.: Photo-oxidation of dimethysulphide in aqueous solution, Mar. Chem., 19, 343–353, 1986.
Brugger, A., Slezak, D., Obernosterer, I., and Herndl, G. J.: Photolysis of dimethylsulfide in the northern Adriatic Sea: Dependence on substrate concentration, irradiance and DOC concentration, Mar. Chem., 59, 321–331, https://doi.org/10.1016/S0304-4203(97)00090-X, 1998.
Carlucci, A., Craven, D., and Henrichs, S.: Surface-film microheterotrophs: amino acid metabolism and solar radiation effects on their activities, Mar. Biol., 85, 13–22, 1985.
Charlson, R. J., Lovelock, J. E., Andreae, M. O., and Warren, S. G.: Oceanic phytoplankton, atmospheric sulphur, cloud albedo and climate, Nature, 326, 655–661, 1987.
Conrad, R. and Seiler, W.: Influence of the Surface Microlayer on the Flux of Nonconservative Trace Gases (CO, H2, CH4, N2O) Across the Ocean-Atmosphere Interface, J. Atmos. Chem., 6, 83–94, 1988.
Cunliffe, M., Upstill-Goddard, R. C., and Murrell, J. C.: Microbiology of aquatic surface microlayers, FEMS Microbiol. Rev., 35, 233–246, https://doi.org/10.1111/j.1574-6976.2010.00246.x, 2011.
Cunliffe, M., Engel, A., Frka, S., Gašparoviæ, B., Guitart, C., Murrell, J. C., Salter, M., Stolle, C., Upstill-Goddard, R., and Wurl, O.: Sea surface microlayers: A unified physicochemical and biological perspective of the air–ocean interface, Prog. Oceanogr., 109, 104–116, https://doi.org/10.1016/j.pocean.2012.08.004, 2013.
Curson, A. R., Liu, J., Martínez, A. B., Green, R. T., Chan, Y., Carrión, O., Williams, B. T., Zhang, S.-H., Yang, G.-P., and Page, P. C. B.: Dimethylsulfoniopropionate biosynthesis in marine bacteria and identification of the key gene in this process, Nat. Microbiol., 2, 17009, https://doi.org/10.1038/nmicrobiol.2017.9, 2017.
Dacey, J. W., Howse, F. A., Michaels, A. F., and Wakeham, S. G.: Temporal variability of dimethylsulfide and dimethylsulfoniopropionate in the Sargasso Sea, Deep-Sea Res. Pt. I, 45, 2085–2104, https://doi.org/10.1016/S0967-0637(98)00048-X, 1998.
Frew, N. M., Nelson, R. K., Mcgillis, W. R., Edson, J. B., Bock, E. J., and Hara, T.: Spatial variations in surface microlayer surfactants and their role in modulating air-sea exchange, Washington, D.C., American Geophysical Union Geophysical Monograph Series, 127, 153–159, https://doi.org/10.1029/GM127p0153, 2002.
Frew, N. M., Bock, E. J., Schimpf, U., Hara, T., Haußecker, H., Edson, J. B., McGillis, W. R., Nelson, R. K., McKenna, S. P., and Uz, B. M.: Air-sea gas transfer: Its dependence on wind stress, small-scale roughness, and surface films, J. Geophys. Res.-Ocean., 109, C08S17, https://doi.org/10.1029/2003JC002131, 2004.
Gali, M., Ruiz-Gonzàlez, C., Lefort, T., Gasol, J. M., Cardelús, C., Romera-Castillo, C., and Simó, R.: Spectral irradiance dependence of sunlight effects on plankton dimethylsulfide production, Limnol. Oceanogr., 58, 489–504, https://doi.org/10.4319/lo.2013.58.2.0489, 2013.
Galí, M. and Simó, R.: A meta-analysis of oceanic DMS and DMSP cycling processes: Disentangling the summer paradox, Global Biogeochem. Cy., 29, 496–515, https://doi.org/10.1002/2014GB004940, 2015.
Galí, M., Simó, R., Pérez, G. L., Ruiz-González, C., Sarmento, H., Royer, S. J., Fuentes-Lema, A., and Gasol, J. M.: Differential response of planktonic primary, bacterial, and dimethylsulfide production rates to static vs. dynamic light exposure in upper mixed-layer summer sea waters, Biogeosciences, 10, 7983–7998, https://doi.org/10.5194/bg-10-7983-2013, 2013.
Hardy, J. T.: The sea-surface microlayer – biology, chemistry and anthropogenic enrichment, Prog. Oceanogr., 11, 307–328, 1982.
Hefu, Y. and Kirst, G. O.: Effect of UV-radiation on DMSP content and DMS formation of Phaeocystis antarctica, Polar Biol., 18, 402–409, 1997.
Herndl, G. J., Muller-Niklas, G., and Frick, J.: Major role of ultraviolet-B in controlling bacterioplankton growth in the surface layer of the ocean, Nature, 361, 717–719, https://doi.org/10.1038/361717a0, 1993.
Hunter, K. A.: Processes affecting particulate trace metals in the sea surface microlayer, Mar. Chem., 9, 49–70, https://doi.org/10.1016/0304-4203(80)90006-7, 1980.
Keller, M. D. and Korjeff-Bellows, W.: Physiological aspects of the production of dimethylsulfoniopropionate (DMSP) by marine phytoplankton, in: Biological and environmental chemistry of DMSP and related sulfonium compounds, Springer, Boston, MA, 131–142, https://doi.org/10.1007/978-1-4613-0377-0_12, 1996.
Keller, M. D., Bellows, W. K., and Guillard, R. L.: Dimethyl Sulfide Production in Marine Phytoplankton, Biogenic Sulfur in the Environment, 393, 167–182, https://doi.org/10.1021/bk-1989-0393.ch011, 1989.
Kieber, D. J., Jiao, J., Kiene, R. P., and Bates, T. S.: Impact of dimethylsulfide photochemistry on methyl sulfur cycling in the equatorial Pacific Ocean, J. Geophys. Res.-Ocean., 101, 3715–3722, https://doi.org/10.1029/95jc03624, 1996.
Kiene, R. P.: Production of methanethiol from dimethylsulfoniopropionate in marine surface waters, Mar. Chem., 54, 69–83, https://doi.org/10.1016/0304-4203(96)00006-0, 1996.
Kiene, R. P. and Linn, L. J.: The fate of dissolved dimethylsulfiniopropionate (DMSP) in seawater: Tracer studies using 35S-DMSP, Geochim. Cosmochim. Ac., 64, 2797–2810, https://doi.org/10.1016/S0016-7037(00)00399-9, 2000.
Lana, A., Bell, T., Simó, R., Vallina, S., Ballabrera-Poy, J., Kettle, A., Dachs, J., Bopp, L., Saltzman, E., and Stefels, J.: An updated climatology of surface dimethlysulfide concentrations and emission fluxes in the global ocean, Global Biogeochem. Cy., 25, GB1004, https://doi.org/10.1029/2010GB003850, 2011.
Leaitch, W. R., Sharma, S., Huang, L., Toom-Sauntry, D., Chivulescu, A., Macdonald, A. M., von Salzen, K., Pierce, J. R., Bertram, A. K., Schroder, J. C., Shantz, N. C., Chang, R. Y.-W., and Norman, A.-L.: Dimethyl sulfide control of the clean summertime Arctic aerosol and cloud, Elementa, Science of the Anthropocene, 1, 000017, https://doi.org/10.12952/journal.elementa.000017, 2013.
Leck, C. and Bigg, E. K.: Source and evolution of the marine aerosol – A new perspective, Geophys. Res. Lett., 32, L19803, https://doi.org/10.1029/2005GL023651, 2005.
Lizotte, M., Levasseur, M., Law, C. S., Walker, C. F., Safi, K. A., Marriner, A., and Kiene, R. P.: Dimethylsulfoniopropionate (DMSP) and dimethyl sulfide (DMS) cycling across contrasting biological hotspots of the New Zealand subtropical front, Ocean Sci., 13, 961–982, https://doi.org/10.5194/os-13-961-2017, 2017.
Matrai, P. A., Tranvik, L., Leck, C., and Knulst, J. C.: Are high Arctic surface microlayers a potential source of aerosol organic precursors?, Mar. Chem., 108, 109–122, https://doi.org/10.1016/j.marchem.2007.11.001, 2008.
Miles, C. J., Bell, T. G., and Lenton, T. M.: Testing the relationship between the solar radiation dose and surface DMS concentrations using in situ data, Biogeosciences, 6, 1927–1934, https://doi.org/10.5194/bg-6-1927-2009, 2009.
Muller-Niklas, G., Heissenberger, A., Stasa, P., and Herndl, G. J.: Ultraviolet-B radiation and bacterial metabolism in coastal waters, Aquat. Microb. Ecol., 9, 111–116, https://doi.org/10.3354/ame009111, 1995.
Nguyen, B. C., Gaudry, A., Bonsang, B., and Lambert, G.: Reevaluation of the role of dimethyl sulphide in the sulphur budget, Nature, 275, 637–639, https://doi.org/10.1038/275637a0, 1978.
Ortega-Retuerta, E., Passow, U., Duarte, C. M., and Reche, I.: Effects of ultraviolet B radiation on (not so) transparent exopolymer particles, Biogeosciences, 6, 3071–3080, https://doi.org/10.5194/bg-6-3071-2009, 2009.
Park, K. T., Jang, S., Lee, K., Yoon, Y. J., Kim, M. S., Park, K., Cho, H. J., Kang, J. H., Udisti, R., Lee, B. Y., and Shin, K. H.: Observational evidence for the formation of DMS-derived aerosols during Arctic phytoplankton blooms, Atmos. Chem. Phys., 17, 9665–9675, https://doi.org/10.5194/acp-17-9665-2017, 2017.
Quinn, P. K. and Bates, T. S.: The case against climate regulation via oceanic phytoplankton sulphur emissions, Nature, 480, 51–56, https://doi.org/10.1038/nature10580, 2011.
Reinthaler, T., Sintes, E., and Herndl, G. J.: Dissolved organic matter and bacterial production and respiration in the sea-surface microlayer of the open Atlantic and western Mediterranean Sea, Limnol. Oceanogr., 53, 122–136, https://doi.org/10.4319/lo.2008.53.1.0122, 2008.
Rellinger, A. N., Kiene, R. P., del Valle, D. A., Kieber, D. J., Slezak, D., Harada, H., Bisgrove, J., and Brinkley, J.: Occurrence and turnover of DMSP and DMS in deep waters of the Ross Sea, Antarctica, Deep-Sea Res. Pt. I, 56, 686–702, https://doi.org/10.1016/j.dsr.2008.12.010, 2009.
Roslan, R. N., Hanif, N. M., Othman, M. R., Azmi, W. N., Yan, X. X., Ali, M. M., Mohamed, C. A., and Latif, M. T.: Surfactants in the sea-surface microlayer and their contribution to atmospheric aerosols around coastal areas of the Malaysian peninsula, Mar. Pollut. Bull., 60, 1584–1590, https://doi.org/10.1016/j.marpolbul.2010.04.004, 2010.
Saint-Macary, A. D., Marriner, A., Barthelmeß, T., Deppeler, S., Safi, K., Costa Santana, R., Harvey, M., and Law, C. S.: DMS cycling in the Sea Surface Microlayer in the South West Pacific: 1. Enrichment potential determined using a novel sampler, EGUsphere [preprint], https://doi.org/10.5194/egusphere-2022-499, 2022.
Sanchez, K. J., Chen, C.-L., Russell, L. M., Betha, R., Liu, J., Price, D. J., Massoli, P., Ziemba, L. D., Crosbie, E. C., Moore, R. H., Müller, M., Schiller, S. A., Wisthaler, A., Lee, A. K. Y., Quinn, P. K., Bates, T. S., Porter, J., Bell, T. G., Saltzman, E. S., Vaillancourt, R. D., and Behrenfeld, M. J.: Substantial Seasonal Contribution of Observed Biogenic Sulfate Particles to Cloud Condensation Nuclei, Sci. Rep., 8, 3235, https://doi.org/10.1038/s41598-018-21590-9, 2018.
Schlitzer, R.: Ocean Data View, https://odv.awi.de (last access: 13 October 2022), 2020.
Sieburth, J.: Microbiological and Organic-Chemical Processes in the Surface and Mixed Layers, in: Air-Sea Exchange of Gases and Particles, edited by: Liss, P. S. and Slinn, W. G. N., NATO ASI Series, Vol. 108, Springer, Dordrecht, 121–172, https://doi.org/10.1007/978-94-009-7169-1_3, 1983.
Simó, R. and Dachs, J.: Global ocean emission of dimethylsulfide predicted from biogeophysical data, Global Biogeochem. Cy., 16, 1078, https://doi.org/10.1029/2001gb001829, 2002.
Simó, R. and Pedrós-Alió, C.: Short-term variability in the open ocean cycle of dimethylsulfide, Global Biogeochem. Cy., 13, 1173–1181, https://doi.org/10.1029/1999gb900081, 1999.
Simó, R., Pedrós-Alió, C., Malin, G., and Grimalt, J. O.: Biological turnover of DMS, DMSP and DMSO in contrasting open-sea waters, Mar. Ecol. Prog. Ser., 203, 1–11, https://doi.org/10.3354/meps203001, 2000.
Slezak, D., Brugger, A., and Herndl, G. J.: Impact of solar radiation on the biological removal of dimethylsulfoniopropionate and dimethylsulfide in marine surface waters, Aquat. Microb. Ecol., 25, 87–97, https://doi.org/10.3354/ame025087, 2001.
Slezak, D., Kiene, R. P., Toole, D. A., Simó, R., and Kieber, D. J.: Effects of solar radiation on the fate of dissolved DMSP and conversion to DMS in seawater, Aquat. Sci., 69, 377–393, https://doi.org/10.1007/s00027-007-0896-z, 2007.
Stefels, J.: Physiological aspects of the production and conversion of DMSP in marine algae and higher plants, J. Sea Res., 43, 183–197, https://doi.org/10.1016/S1385-1101(00)00030-7, 2000.
Stefels, J., Steinke, M., Turner, S., Malin, G., and Belviso, S.: Environmental constraints on the production and removal of the climatically active gas dimethylsulphide (DMS) and implications for ecosystem modelling, Biogeochemistry, 83, 245–275, https://doi.org/10.1007/s10533-007-9091-5, 2007.
Stolle, C., Ribas-Ribas, M., Badewien, T. H., Barnes, J., Carpenter, L. J., Chance, R., Damgaard, L. R., Durán Quesada, A. M., Engel, A., Frka, S., Galgani, L., Gašparoviæ, B., Gerriets, M., Hamizah Mustaffa, N. I., Herrmann, H., Kallajoki, L., Pereira, R., Radach, F., Revsbech, N. P., Rickard, P., Saint, A., Salter, M., Striebel, M., Triesch, N., Uher, G., Upstill-Goddard, R. C., van Pinxteren, M., Zäncker, B., Zieger, P., and Wurl, O.: The MILAN Campaign: Studying Diel Light Effects on the Air–Sea Interface, Bull. Am. Meteorol. Soc., 101, E146–E166, https://doi.org/10.1175/bams-d-17-0329.1, 2020.
Sunda, W. G., Kieber, D. J., Kiene, R. P., and Huntsman, S.: An antioxidant function for DMSP and DMS in marine algae, Lett. Nat., 418, 317–320, https://doi.org/10.1038/nature00851, 2002.
Toole, D., Slezak, D., Kiene, R., Kieber, D., and Siegel, D.: Effects of solar radiation on dimethylsulfide cycling in the western Atlantic Ocean, Deep-Sea Res. Pt. I, 53, 136–153, https://doi.org/10.1016/j.dsr.2005.09.003, 2006a.
Toole, D. A., Slezak, D., Kiene, R. P., Kieber, D. J., and Siegel, D. A.: Effects of solar radiation on dimethylsulfide cycling in the western Atlantic Ocean, Deep-Sea Res. Pt. I, 53, 136–153, https://doi.org/10.1016/j.dsr.2005.09.003, 2006b.
Upstill-Goddard, R. C., Frost, T., Henry, G. R., Franklin, M., Murrell, J. C., and Owens, N. J.: Bacterioneuston control of air-water methane exchange determined with a laboratory gas exchange tank, Global Biogeochem. Cy., 17, 1108, https://doi.org/10.1029/2003GB002043, 2003.
Vallina, S. M. and Simó, R.: Strong relationship between DMS and the solar radiation dose over the global surface ocean, Science, 315, 506–508, https://doi.org/10.1126/science.1133680, 2007.
van Rijssel, M. and Buma, A. G.: UV radiation induced stress does not affect DMSP synthesis in the marine prymnesiophyte Emiliania huxleyi, Aquat. Microb. Ecol., 28, 167–174, https://doi.org/10.3354/ame028167, 2002.
Vila-Costa, M., Kiene, R. P., and Simí, R.: Seasonal variability of the dynamics of dimethylated sulfur compounds in a coastal northwest Mediterranean site, Limnol. Oceanogr., 53, 198–211, https://doi.org/10.4319/lo.2008.53.1.0198, 2008.
Vogt, M. and Liss, P.: Dimethylsulfide and climate, Surface Ocean-Lower Atmosphere Processes, edited by: Le Quéré, C., and Saltzman, ES, American Geophysical Union, Washington, DC, 197–232, https://doi.org/10.1029/2008GM000790, 2009.
Walker, C. F., Harvey, M. J., Smith, M. J., Bell, T. G., Saltzman, E. S., Marriner, A. S., McGregor, J. A., and Law, C. S.: Assessing the potential for dimethylsulfide enrichment at the sea surface and its influence on air-sea flux, Ocean Sci., 12, 1033–1048, https://doi.org/10.5194/os-12-1033-2016, 2016.
Wang, W.-L., Song, G., Primeau, F., Saltzman, E. S., Bell, T. G., and Moore, J. K.: Global ocean dimethyl sulfide climatology estimated from observations and an artificial neural network, Biogeosciences, 17, 5335–5354, https://doi.org/10.5194/bg-17-5335-2020, 2020.
Wolfe, G. V. and Kiene, R. P.: Effects of methylated, organic, and inorganic substrates on microbial consumption of dimethyl sulfide in estuarine waters, Appl. Environ. Microbiol., 59, 2723–2726, https://doi.org/10.1128/aem.59.8.2723-2726.1993, 1993.
Yang, G.-P.: Dimethylsulfide enrichment in the surface microlayer of the South China Sea, Mar. Chem., 66, 215–224, https://doi.org/10.1016/S0304-4203(99)00042-0, 1999.
Yang, G.-P. and Tsunogai, S.: Biogeochemistry of dimethylsulfide (DMS) and dimethylsulfoniopropionate (DMSP) in the surface microlayer of the western North Pacific, Deep-Sea Res. Pt. I, 52, 553–567, https://doi.org/10.1016/j.dsr.2004.11.013, 2005.
Yang, G.-P., Tsunogai, S., and Watanabe, S.: Biogenic sulfur distribution and cycling in the surface microlayer and subsurface water of Funka Bay and its adjacent area, Cont. Shelf Res., 25, 557–570, https://doi.org/10.1016/j.csr.2004.11.001, 2005a.
Yang, G.-P., Watanabe, S., and Tsunogai, S.: Distribution and cycling of dimethylsulfide in surface microlayer and subsurface seawater, Mar. Chem., 76, 137–153, https://doi.org/10.1016/S0304-4203(01)00054-8, 2001.
Yang, G.-P., Levasseur, M., Michaud, S., and Scarratt, M.: Biogeochemistry of dimethylsulfide (DMS) and dimethylsulfoniopropionate (DMSP) in the surface microlayer and subsurface water of the western North Atlantic during spring, Mar. Chem., 96, 315–329, https://doi.org/10.1016/j.marchem.2005.03.003, 2005b.
Yang, G.-P., Levasseur, M., Michaud, S., Merzouk, A., Lizotte, M., and Scarratt, M.: Distribution of dimethylsulfide and dimethylsulfoniopropionate and its relation with phytoneuston in the surface microlayer of the western North Atlantic during summer, Biogeochemistry, 94, 243–254, https://doi.org/10.1007/s10533-009-9323-y, 2009.
Yoch, D. C.: Dimethylsulfoniopropionate: Its sources, role in the marine food web, and biological degradation to dimethylsulfide, Appl. Environ. Microbiol., 68, 5804–5815, https://doi.org/10.1128/AEM.68.12.5804-5815.2002, 2002.
Yu, F. and Luo, G.: Oceanic Dimethyl Sulfide Emission and New Particle Formation around the Coast of Antarctica: A Modeling Study of Seasonal Variations and Comparison with Measurements, Atmosphere, 1, 34–50, https://doi.org/10.3390/atmos1010034, 2010.
Zemmelink, H. J., Houghton, L., Sievert, S. M., Frew, N. M., and Dacey, J. W.: Gradients in dimethylsulfide, dimethylsulfoniopropionate, dimethylsulfoxide, and bacteria near the sea surface, Mar. Ecol. Prog. Ser., 295, 33–42, https://doi.org/10.3354/meps295033, 2005.
Zemmelink, H. J., Houghton, L., Frew, N. M., and Dacey, J. W. H.: Dimethylsulfide and major sulfur compounds in a stratified coastal salt pond, Limnol. Oceanogr., 51, 271–279, https://doi.org/10.4319/lo.2006.51.1.0271, 2006.
Zhang, H.-H., Yang, G.-P., and Zhu, T.: Distribution and cycling of dimethylsulfide (DMS) and dimethylsulfoniopropionate (DMSP) in the sea-surface microlayer of the Yellow Sea, China, in spring, Cont. Shelf Res., 28, 2417–2427, https://doi.org/10.1016/j.csr.2008.06.003, 2008.
Zhang, H.-H., Yang, G.-P., Liu, C.-Y., and Li, C.: Seasonal variations od dimethylsulfide (DMS) and dimethylsulfoniopropionate (DMSP) in the sea-surface microlayer and subsurface water of Jiaozhou Bay and its adjacent area, Acta Oceanol. Sin., 28, 73–86, 2009.