the Creative Commons Attribution 4.0 License.
the Creative Commons Attribution 4.0 License.
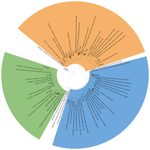
Salinity as a key control on the diazotrophic community composition in the southern Baltic Sea
Christian Furbo Reeder
Ina Stoltenberg
Jamileh Javidpour
Carolin Regina Löscher
Over the next decade, the Baltic Sea is predicted to undergo severe changes including decreased salinity due to altered precipitation related to climate changes. This will likely impact the distribution and community composition of Baltic Sea dinitrogen-fixing (N2-fixing) microbes, among which heterocystous cyanobacteria are especially adapted to low salinities and may expand to waters with currently higher salinity, including the Danish Strait and Kattegat, while other high-salinity-adapted N2 fixers might decrease in abundance.
In order to explore the impact of salinity on the distribution and activity of different diazotrophic clades, we followed the natural salinity gradient from the eastern Gotland and Bornholm basins through the Arkona Basin to the Kiel Bight and combined N2 fixation rate measurements with a molecular analysis of the diazotrophic community using the key functional marker gene for N2 fixation nifH, as well as the key functional marker genes anfD and vnfD, encoding for the two alternative nitrogenases.
We detected N2 fixation rates between 0.7 and 6 nmol N L−1 d−1, and the diazotrophic community was dominated by the cyanobacterium related to Nodularia spumigena and the small unicellular, cosmopolitan cyanobacterium UCYN-A. Nodularia was present in gene abundances between 8.07 × 105 and 1.6 × 107 copies L−1 in waters with salinities of 10 and below, while UCYN-A reached gene abundances of up to 4.5 × 107 copies L−1 in waters with salinity above 10. Besides those two cyanobacterial diazotrophs, we found several clades of proteobacterial N2 fixers and alternative nitrogenase genes associated with Rhodopseudomonas palustris, a purple non-sulfur bacterium. Based on principal component analysis (PCA), salinity was identified as the primary parameter describing the diazotrophic distribution, while pH and temperature did not have a significant influence on the diazotrophic distribution. While this statistical analysis will need to be explored in direct experiments, it gives an indication for the future development of diazotrophy in a freshening Baltic Sea with UCYN-A retracting to more saline North Sea waters and heterocystous cyanobacteria expanding as salinity decreases.
- Article
(4291 KB) - Full-text XML
-
Supplement
(469 KB) - BibTeX
- EndNote
The Baltic Sea (Fig. 1) is a marginal, brackish sea characterized by a natural salinity gradient increasing from the northeast to the southwest. The Baltic Sea covers an area of 415 000 km2 with a permanent halocline in the Baltic Sea Proper, preventing vertical mixing, and oxygen (O2)-depleted waters in the deeper basins and coastal systems, accompanied by the occasional accumulation of hydrogen sulfide (H2S) and ammonium (NH) below the chemocline (Conley et al., 2002; Hietanen et al., 2012; Lennartz et al., 2014). It is further challenged by a high land-derived influx of phosphorus, leading to a substantial internal surface water and sedimentary phosphorus load (Gustafsson et al., 2017; Stigebrandt and Andersson, 2020). Prior studies have shown that the Baltic Sea experienced a 10-fold increase in O2-depleted areas over the last 115 years, covering an area of 12 000–70 000 km2, making it one of the ocean areas most severely affected by deoxygenation (Diaz and Rosenberg, 2008; Reusch et al., 2018). Between 1871 and 2013, the Baltic Sea showed an increase in temperature of 0.1 ∘C decade−1, exceeding the average global trend of about 0.06 ∘C decade−1 (Reusch et al., 2018; Rutgersson et al., 2014), and decreased salinity (1–2 kg−1) (Liblik and Lips, 2019). Nitrogen (N) deposition rates are now among the highest in marine areas (Reusch et al., 2018). The resulting eutrophication severely affects sensitive coastal areas, resulting in high pelagic production, frequent events of anoxia, and decreased biodiversity (Breitburg et al., 2018; Carstensen et al., 2014; Maar et al., 2016; Reusch et al., 2018; Rutgersson et al., 2014). In this context, the Baltic Sea has been described as a “time machine” for how future oceans will respond to climate change (Reusch et al., 2018), making it an ideal environment to investigate biological fixation of dinitrogen gas (N2 fixation) in response to such changes.
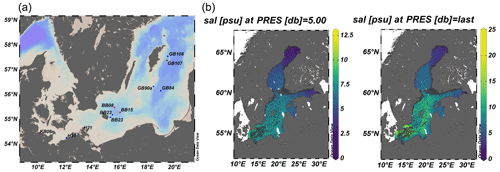
Figure 1Overview of cruise track and stations from this study. (a) Distribution of O2, salinity (sal) in surface water (5 m), and the deepest sample at the station (last) in the Baltic Sea. Stations from which N2 fixation were obtained are colored red. Data for (b) were obtained through ICES oceanography spanning from 1 August to 30 October 2019 (ICES Copenhagen, 2020).
N2 fixation is the primary external source of new N to life in the ocean and plays a crucial role for primary production and thus carbon dioxide (CO2) uptake from the atmosphere (Carpenter and Capone, 2008; Gruber, 2005). Specialized organisms, called diazotrophs, carry out this highly energy-demanding process. The diversity of diazotrophs is not easy to describe, as those organisms spread across the microbial tree of life and are found in both the bacterial and archaeal domains (Zehr et al., 1998). N2 fixation is catalyzed by an enzyme complex, the nitrogenase, which exists in three different subtypes: the molybdenum (Mo) iron (Fe) type referred to as Nif, the vanadium (V) Fe type called Vnf, and an Fe–Fe type called Anf (Chisnell et al., 1988; Robson et al., 1986). The different metal cofactors are essential in the context of the redox sensitivity of the three nitrogenases. Mo is preferentially available in the presences of at least traces of O2 contrary to V and Fe, which are more soluble and thus available under anoxic conditions (Bennett and Canfield, 2020; Bertine and Turekian, 1973; Crusius et al., 1996; Dixon and Kahn, 2004; Morford and Emerson, 1999). The differential availability of those trace metals along redox gradients might have played a role in the evolutionary development of those nitrogenase types (Anbar, 2008; Mus et al., 2019), and their distribution might change in a future ocean impacted by acidification, deoxygenation (Keeling et al., 2010; Löscher et al., 2014; Schmidtko et al., 2017; Stramma et al., 2008), and desalination (Olofsson et al., 2020). To date, most studies focused mainly on Nif-type nitrogenases in the marine environment, while Vnf and Anf nitrogenases were often overlooked. Molecular detection of the nifH gene does not fully recover the diversity of vnfH and anfH, possibly resulting in an underestimation of alternative nitrogenases in the environment (Affourtit et al., 2001; Farnelid et al., 2009, 2013; Man-Aharonovich et al., 2007; Steward et al., 2004; Zehr et al., 1995). Nonetheless, the few available studies focusing on alternative nitrogenases identified them to be abundant and diverse and suggested them to play a role for N2 fixation in various ecosystems, including the upper water column of the ocean, O2-depleted waters, and Baltic Sea sediments (Bellenger et al., 2011, 2014; Betancourt et al., 2008; Christiansen and Löscher, 2019; Farnelid et al., 2009; Loveless et al., 1999; McRose et al., 2017; Tan et al., 2009; Zehr et al., 2003; Zhang et al., 2016).
In the Baltic Sea, N2 fixation is considered to mainly take place in sunlit surface waters carried out by three genera of heterocystous cyanobacteria: Aphanizomenon spp., N. spumigena, and Anabaena spp. (now referred to as Dolichosperum spp. (Klawonn et al., 2016; Wacklin et al., 2009), all of them containing the classic Nif nitrogenase (Janson et al., 1994; Leppanen et al., 1988) and contributing massively to N2 fixation with rates of up to 115–295 nmol N L−1 d−1 (Larsson et al., 2001). At the same time, cyanobacteria consume only a fraction of the N they fix with estimates in the range of 33.33–85.72 nmol N L−1 d−1 (Janson et al., 1994; Larsson et al., 2001; Wasmund, 1997). Using an approach based on elemental analyzer–isotope ratio mass spectrometry (EA-IRMS), Klawonn et al. (2016) measured N2 fixation rates up to 300 ± 216 nmol N L−1 d−1 and, based on cell-specific measurement of Aphanizomenon spp. and N. spumigena, revealed that they release up to 35 % of fixed N, translating into a substantial fraction of fixed N leaking out available for other primary producers (Ploug et al., 2010, 2011). In addition, low rates of N2 fixation (0.44 nmol N L−1 d−1) have been described in anoxic waters of the Baltic Sea (Farnelid et al., 2013). These rates were accompanied by a highly diverse Nif-containing heterotrophic diazotroph community at and below the chemocline in anoxic NH-rich waters (Farnelid et al., 2013). Heterotrophic diazotrophs were closely related to those in other O2-depleted marine system regions including the eastern tropical South (ETSP) and North Pacific (ETNP), the Arabian Sea (AS), and an anoxic basin in the Californian Bight (Fernandez et al., 2011; Hamersley et al., 2011; Jayakumar et al., 2012, 2017; Löscher et al., 2014). Non-cyanobacterial diazotrophs have been found to fix N2 actively. However, it is still inconclusive what regulates and controls their N2 fixation activity.
Over the last 3 decades, the Baltic Sea has experienced a trend of freshening in the range of 1–2 kg−1 in surface waters (Liblik and Lips, 2019) or between 0.4 and 1.2 (Saraiva et al., 2019). Earlier studies suggested that salinity impacts the diazotrophic community composition and the activity of nitrogenases in pure cultures of Azotobacter sp. marine microbial mats and estuaries (Dicker and Smith, 1981; Marino et al., 2006; Severin et al., 2012). Surface salinity in the Baltic Sea ranges between 0 and 10 (Fig. 1), making it one of the major drivers for microbial composition and distribution in the Baltic Sea (Dupont et al., 2014; Olofsson et al., 2020; Wulff et al., 2018). Typically, Aphanizomenon spp. dominate less saline waters (e.g., Bothnian Sea) and grow best with salinities around 5 (Lehtimäki et al., 1997). Aphanizomenon spp., however, have also been frequently reported to grow at salinities reaching up to 5–8 such as in the northern Baltic Proper (Olofsson et al., 2020). N. spumigena prefers the higher-salinity waters in the southern part (e.g., the southern Baltic Proper) with an optimum growth in salinities of 8–10 (Lehtimäki et al., 1997; Rakko and Seppälä, 2014). Additionally, the small, unicellular cyanobacterial symbiont UCYN-A has been detected in the Baltic Sea (Bentzon-Tilia et al., 2015). This cosmopolitan diazotroph has previously been shown to be abundant throughout most marine systems (Tang et al., 2019; Zehr et al., 2016) and to substantially contribute to N2 fixation rates (Martínez-Pérez et al., 2016; Mills et al., 2020). However, the factors controlling the biogeography of UCYN-A are poorly understood. However, the latest study suggested salinity as having a positive correlation with UCYN-A biogeography (Li et al., 2021). These observations indicate potential changes in the cyanobacterial composition in future freshening events (Olofsson et al., 2020; Wasmund et al., 2011). To now explore the impact and importance of salinity for diazotrophy in the Baltic Sea and in order to complement existing datasets on N2 fixation from high-productivity seasons, we carried out a survey including chemical profiling and rate measurements in the low-productivity fall season. The focus of this study is a molecular genetic assessment of the diazotrophic community diversity and genetic activity including heterocyst and unicellular cyanobacteria, heterotroph diazotrophs, and diazotrophs carrying alternative nitrogenase genes. In addition, we compared our data to those available to be able to predict the future distribution of Baltic Sea diazotrophs and N2 fixation rates.
2.1 Seawater sampling
Samples were collected during the cruise AL528 using the German research vessel RV Alkor from 17 to 28 September 2019 along a transect through the major basins of the Baltic Sea (see Fig. 1 for a cruise plan and Table S2 for an overview of all stations). Specifically, samples were collected at a station in the Kiel Fjord (KB06), one in the Arkona Basin (H21), four stations in the Bornholm Basin (BB08, BB15, BB23, BB31), and four in the eastern Gotland Basin (GB84, GB90a, GB107, GB108) at water depths between 3 and 115 m (m) using a 10 L Niskin bottle rosette equipped with a conductivity–temperature–depth (CTD) sensor. Water for dissolved inorganic nitrogen NOx (nitrate (NO) + nitrite (NO)) was collected from the Niskin bottles, filtered through an acid-washed 0.8 µm cellulose acetate filter (Sartorius™) using a syringe, and stored in 20 mL scintillation vials at −20 ∘C until analysis. Samples for PO analysis were filtered through a 200 µm mesh to avoid contamination with large zooplankton. Samples were filled in 20 mL scintillation vials and stored at −80 ∘C until analysis. NOx and PO samples were analyzed on a SKALAR SANplus analyzer (Thermo Fisher, Waltham, US) according to Grasshoff (1999) with a detection limit of 0.05 µmol N L−1 for NOx and 0.03 µmol P L−1 for PO. Nutrient samples were taken in triplicates and handled with nitrile gloves, and all equipment was rinsed with Milli-Q water between stations to avoid contamination. Samples for dissolved inorganic carbon (DIC) were collected by filling triplicates of 12 mL Exetainers bubble-free at stations KB06, H31, H21, and BB15. Samples were fixed with 20 µL of a saturated mercury chloride solution and stored at room temperature until analysis. DIC measurements were carried out using a 2 mM NaHCO3 solution as standard and as described previously (Hall and Aller, 1992). From all stations and depths 0.5 to 1 L of seawater was collected from Niskin bottles and immediately filtered onto 0.22 µm pore size membrane filters (Millipore, Bilaterica, USA; exact seawater volumes were constantly recorded); filters were stored at −80 ∘C until DNA extraction and further analysis.
2.2 15N2 and 13C seawater incubations
N2 and C fixation rates were determined using stable isotope labeling at KB06, H21, BB15, BB08, and GB107 at water depths between 3 and 41 m. We used the bubble addition method (Montoya et al., 1996) to ensure comparability to previous studies from the Baltic Sea. Triplicate water samples were filled from Niskin bottles into 2.4 L glass bottles until top-filled (Schott-Duran, Wertheim, Germany). To each incubation bottle, 1 mL 15N2 gas (15N2 98 % + lot no. I-16727, Cambridge Isotope Laboratories, Inc., USA) and 1 mL H13CO3 (1 g 50 mL−1 Sigma-Aldrich, Saint Louis Missouri US) was added through an airtight septum, and bottles were inverted to ensure mixing with final calculated concentrations of 0.8 atom % 15N2 and 10 µg mL−1 H13CO3 (approximately 3.8 atom %). After an incubation time of 24 h, samples were filtered onto pre-combusted GF/F filters (GE Healthcare Life Sciences, Whatman, USA). A parallel set of triplicate samples was collected from each sampling depth for determining the natural abundance of N and C isotopes in the particulate organic material. Filters were stored at −20 ∘C until further analysis. Filters were then acidified and dried in an oven at 65 ∘C. Dried filters were analyzed on an Elemental Analyzer Flash EA 1112 series (Thermo Fisher) coupled to an isotope ratio mass spectrometer (Finnigan Delta Plus XP, Thermo Fisher).
2.3 Molecular methods
For nucleic acid extraction, 0.22 µm pore size Millipore membrane filters (Millipore, Bilaterica, USA) were flash-frozen in liquid N and subsequently crushed. DNA was purified with the MasterPure Complete DNA and RNA purification Kit (Lucigen, Wisconsin, US) according to the manufacturer's protocol, with the minor modification of using 1 mL of lysis buffer to completely cover the filter pieces. RNA was purified using the Qiagen AllPrep DNA/RNA Mini Kit (Qiagen, Hilden, Germany). The remaining DNA was removed with the gDNA wipeout mix, and a cDNA library was constructed using the QuantiTect Reverse Transcription Kit (Qiagen, Hilden, Germany) with the supplied RT primer set. The nucleic acid concentration and quality were checked spectrophotometrically on a MySpec spectrofluorometer (VWR, Darmstadt, Germany).
A total of 15 DNA samples were amplified for nifH using nested PCR with primers and PCR conditions as described in Zani et al. (2000). The anfD and vnfD were amplified using a nested PCR with primers and conditions as described in Bellenger et al. (2014) and McRose et al. (2017). Amplicons were TOPO TA-cloned (Topo TA cloning Kit for sequencing, Thermo Fisher Scientific, Waltham, US) and Sanger-sequenced. Sequencing was carried out at the Institute of Clinical Molecular Biology in Kiel, Germany. Samples for nifH and anfD/vnfD amplification were the same as the ones for which N2 rate measurements were carried out (KB06, H21, BB15, BB08, and GB107 between 3 and 41 m; see Table S1).
Sequences were quality-checked and trimmed with BioEdit (Hall, 1999). The nifH sequences were trimmed to 321 bp and anfD/vnfD to 591 bp. This resulted in 182 nifH and 69 anfD/vnfD amplicon sequences. Sequences were BLASTX-searched against the NCBI database. A reference library was created, and sequences with lower than 80 % identity were discarded. Sequences were aligned in MEGAX (Kumar et al., 2018), and a maximum likelihood tree was constructed using 1000 bootstraps. To explore the overall microbial diversity, 23 samples from Kiel Fjord (station KB06, depth 5 and 10 m), Arkona Basin (station H2, depth 4, 12, 42 m; station H31, depth 4 and 12 m), Bornholm Basin (station BB23, depth 4, 48, 85 m; station BB15, depth 7, 41, 65 m; station BB08, depth 5 and 10 m), and eastern Gotland Basin (station GB84, depth 9, 29, 110 m; station GB107, depth 8, 40, and 112 m; station GB108, depth 10 and 115 m) were sent to amplicon sequencing of the 16s rDNA V1V2 region using Illumina HiSeq technology. Depths were chosen based on the water column chemistry (halocline, oxycline, oxygen minimum zones, and chlorophyll peaks). Sequencing was carried out by the Institute of Clinical Molecular Biology in Kiel, Germany. Raw reads were trimmed, quality-filtered in dada2 (v1.18) (Callahan et al., 2016), and taxonomically annotated using the SILVA database (v138) (Quast et al., 2013). Visualization of the cyanobacterial distribution was accessed with phyloseq (1.34.0) (McMurdie and Holmes, 2013).
Quantitative real-time PCRs were carried out targeting the nifH clade specifically for Nodularia, UCYN-A, and Gammaproteobacterial diazotrophs (gamma AO, gamma PO) as described previously (e.g., Boström et al., 2007; Langlois et al., 2008; Löscher et al., 2014). As standards, serial dilutions of plasmids (107 to 101 copies) containing the target nifH genes were used. Samples, standards, and non-template controls were run in duplicates on a Biorad qPCR machine (Biorad, Hercules, USA), and reactions were considered uncontaminated if no amplification could be detected in non-template controls after 40 qPCR cycles. To further ensure that no DNA contamination was present in our cDNA, we ran additional qPCR reactions of RNA samples. No amplification was observed. Sequences were submitted to GenBank with accession numbers MZ063808-MZ063873 and MZ063874-MZ064057 and 16S rDNA with accession number SAMN20309768-SAMN20309783.
2.4 Statistical methods
Principal component analyses (PCAs) on qPCR and environmental data were performed in R using the vegan package (Oksanen et al., 2020; R Foundation for Statistical Computing, 2017). The simper function (from the vegan package) was used to identify parameters with the highest impact on diazotroph abundance. A principal component analysis (PCA) was subsequently performed using prcomp and visualized with the factoextra package (Kassambara and Mundt, 2020). To maximize the amount of explained variance of the dataset, the figure included component 1 versus 2 (Fig. 8a) and component 1 versus 3 (Fig. 8b).
3.1 Hydrochemistry
During the cruise, surface waters above 40 m water depth exhibited the typical Baltic Sea salinity gradient with salinities decreasing from southwest to northeast (10–24 in the Kiel and Arkona Basin and 7–9 in the Bornholm and eastern Gotland basins), accompanied by lower temperatures of 13–14 ∘C in the Bornholm and eastern Gotland Basin compared to slightly higher temperatures of 15–16 ∘C in the Kiel Fjord and Arkona Basin (Fig. 2). A thermocline was observed at 45 m in the Bornholm Basin and 65 m in the eastern Gotland Basin. The coastal station we sampled in the Arkona Basin showed a similar stratification pattern (Fig. 2) with a salinity of 10 on the surface and 17 below 30 m water depth. This stratification pattern is typical for the region and time of the year and could also be observed from annual data from the International Council for the Exploration of the Sea (ICES Copenhagen, 2020) (Fig. 1b). Surface waters were well-oxygenated with O2 concentrations above 156.31 µmol L−1 in the top 40 m of the water column. Low-O2 water masses with concentrations below 15 µmol L−1 were only observed in the Bornholm Basin in waters deeper than 65 m (Fig. 2).
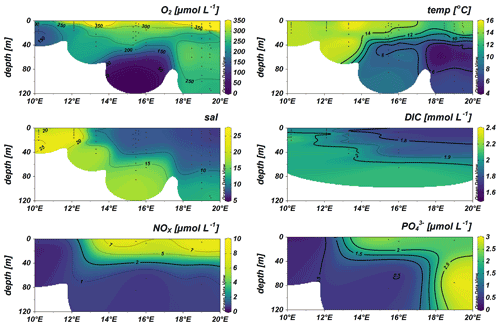
Figure 2Distribution of surface water O2 (µmol L−1), temperature (temp) (∘C), salinity (sal), dissolved inorganic carbon (DIC) (mmol L−1), NOx (nitrate + nitrite), and PO (both µmol L−1).
Compared to the southwestern part, the DIC concentrations were lower in the upper water column of the eastern Gotland and Bornholm Basin, with concentrations of around 1.6 mmol L−1, and increased towards the southwestern part of the cruise track. An opposite trend was visible in nutrient distribution, with NOx (nitrite + nitrate) and phosphate (PO) decreasing from the northeastern part of the cruise track towards the southwestern part and with surface water concentrations of 9.04 µmol L−1 for NOx and 2.98 µmol L−1 for PO in surface waters of the eastern Gotland Basin and 0.27 µmol L−1 for NOx and 0.18 µmol L−1 for PO in the Kiel Fjord (Fig. 2). The observed nutrient concentrations were in the range of the Helsinki commission (HELCOM) dataset containing data from 2015 to 2020 and the HELCOM core indicator report (Helcom, 2018, Fig. S2). The somewhat higher nutrient concentrations detected in the Bornholm and eastern Gotland basins could result from a decaying phytoplankton bloom releasing nutrients including ammonia, decreased microbial activity, or increased eutrophication. High chlorophyll concentrations in the Bornholm Basin (3 µM Chl a in August and 2 µM Chl a in September), derived from the HELCOM dataset (from same areas as this cruise), indeed give evidence of a decaying phytoplankton bloom in the Bornholm Basin in September 2019 (Fig. S3). These values are in range with the HELCOM indicator report, with an average of 4 µM Chl a from June to September, and a previous study presenting a range between 2 and 6 µM Chl a (Helcom, 2018; Suikkanen et al., 2010).
Our dataset indicates an N:P ratio (µmol L−1 : µmol L−1) well below the Redfield ratio of 16:1 in surface waters along the cruise track, with excess P classically assumed to promote N2 fixation. However, NOx was still available to the phytoplankton and microbial community as supported by a positive intercept of the trend line, which might be considered unfavorable for N2 fixation (Fig. 3). Remaining NOx concentrations in the surface waters above 40 m thus speak for a limitation of primary production by either micronutrients, temperature, or light availability and less for a limitation by N or PO (Arrigo, 2005; Saito et al., 2008). Nitrogen repletion is supported by POC : PON ratios of 3.6 to 4.94 in the Kiel Fjord and Arkona Basin and 2.92 to 6.15 in the Bornholm and eastern Gotland basins (Fig. 3) (PON: particulate organic nitrogen, POC: particulate organic carbon), which are close to the Redfield ratio (Redfield, 1934). Altogether, nutrient concentrations do not strongly support a niche for N2 fixation.
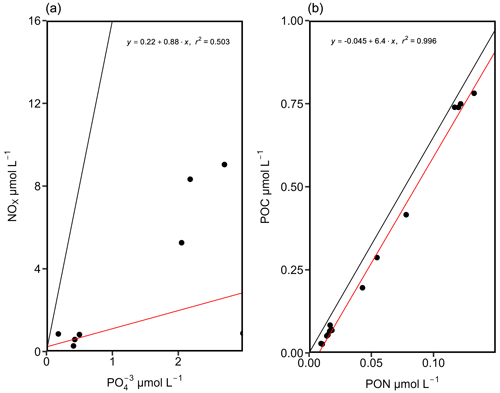
Figure 3N:P (a) and C:N (b) ratios from stations KB06, BB23, BB15, BB08, and GB107. The black lines depict the N:P (16:1) and C:N (106:16) ratios after Redfield. The red lines indicate the trend lines of our data. An N:P ratio below the canonical Redfield ratio of 16:1 suggests a niche for N2 fixation. However, the positive intercept speaks for DIN still being available, which might be considered unfavorable for N2 fixation (a). A C:N ratio below Redfield might suggest a slight deficiency in N (b), and this might result from degrading organic matter in the particulate organic matter (POM) pool.
3.2 Nitrogen fixation
While no clear niche for N2 fixation could be identified, we still detected N2 fixation rates in our samples from water depths between 3 and 41 m (Fig. 4). N2 fixation rates were between 0 and 6 ± 4.73 nmol N L−1 d−1 in the Arkona Basin, 0.7 ± 0.97 to 27.3 ± 38.21 nmol N L−1 d−1 in Bornholm Basin, and 0.67 ± 0.51 to 2 ± 0.4 nmol N L−1 d−1 in the eastern Gotland Basin (Fig. 4). In Kiel Fjord, N2 fixation rates were below the detection limit (0.03 nmol N L−1 d−1) of our method. One outlier was observed in the Bornholm Basin at a water depth of 5 m, with an N2 fixation rate of 27.3 nmol N L−1 d−1. This high rate was only detected in one out of our three replicates and was not included in our further analysis. Without this outlier, a conservative estimate of N2 fixation in the Bornholm Basin would be between 0.7 ± 0.97 and 5.27 ± 1.37 nmol N L−1 d−1. Though these rates are at the lower end of previous observations from the Baltic Sea (Table S3), they are comparable to a previous study from the same region but not the same season, with N2 fixation rates of 7.6 ± 1.76 nmol N L−1 d−1 from July and August (Farnelid et al., 2013). N2 fixation activity is supported by the δ15N–PON in our control samples, which was in the range of control samples and found to be 0.3 ‰ to 2 ‰ in samples from surface waters above 40 m from the eastern Gotland and Bornholm basins (Fig. 4). Values between −2 ‰ and +2 ‰ are considered indicative for N2 fixation (Dähnke and Thamdrup, 2013; Delwiche and Steyn, 1970).
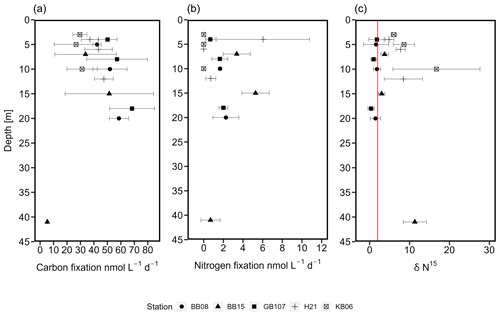
Figure 4C (a) and N2 (b) fixation rates (nmol L−1 d−1). Similarly at all stations (five stations covering three depths), C fixation increased over depth, followed by a decrease at a water depth of 41 m. Higher rates were observed in the Bornholm and eastern Gotland basins compared to the Kiel and Arkona basins. N2 fixation rates increased slightly over depth, followed by a decrease at 41 m water depth in the Bornholm Basin. (c) δ15N (‰) from stations KB06, H21, BB08, BB15, and GB107. The red line indicates a δ15N of 2 ‰. Values below 2 ‰ speak for previous or current N2 fixation (Dähnke and Thamdrup, 2013).
Seasonal variations might explain the observed lower N2 fixation rates if compared to previous studies. N2 fixation rates are generally described as sensitive to low temperatures (Brauer et al., 2013; Church et al., 2009; Englund and Meyerson, 1974; Moisander et al., 2010; Stal, 2009), with the energetic costs of N2 fixation sharply rising at temperatures below 21 ∘C (Brauer et al., 2013). During our cruise, surface temperatures were between 12 and 16 ∘C and would most likely play a role in the low rates observed. Yet, growth of N. spumigena has been demonstrated at 4 ∘C, suggesting relevance during wintertime in the Baltic Sea (Olofsson et al., 2019). Additionally, lower light intensities during fall and winter could directly influence the abundance of cyanobacterial diazotrophs in the Baltic Sea (Dera and Woźniak, 2010; Staal et al., 2002).
N2 fixation rates were accompanied by low C fixation rates compared to rates in the micromolar range as typically detected in the Baltic Sea (e.g., Klawonn et al., 2016) (Fig. 4), which were, however, increasing over depth, with an average C fixation of 29.16 ± 10.89 nmol C L−1 d−1 in Kiel Fjord, 42.62 ± 7.86 nmol C L−1 d−1 the Arkona Basin, 40.52 ± 13.16 nmol C L−1 d−1 in Bornholm Basin, and 58.52 ± 15.42 nmol C L−1 d−1 in eastern Gotland Basin. Assuming Redfield stoichiometry, N2 fixation was sustained between 8.8 % and 108 % with an average of 43 % of C fixation during our cruise. These rates are comparable with the contribution of N2 fixation to C fixation in other oceanic regions, including the Atlantic (20 %–25 %) and Pacific Ocean (1 %–4 %), the Mediterranean Sea (8 %), and the Bay of Bengal (<1 %) (Church et al., 2009; Dore et al., 2002; Fonseca-Batista et al., 2019; Rahav et al., 2013; Saxena et al., 2020; Tang et al., 2019) (Table 1). The high contribution to primary production indicates that N2 fixation may promote or extend primary production into the fall season if only at low rates. Other sources, however, might include land or riverine input.
3.3 Diazotrophic community composition
We assessed the diazotrophic diversity based on nifH and anfD/vnfD amplicons (Figs. 5 and 6). Sequences belonging to N. spumigena (19 %) and UCYN-A (26 %) were dominant in the sequence pool. While Nodularia clades are classically abundant in the Baltic Sea waters (Boström et al., 2007; Farnelid et al., 2013; Klawonn et al., 2016; Larsson et al., 2001), only one previous report of UCYN-A from the Baltic Sea exists; it had been detected in waters of the Danish Strait and the Great Belt (Bentzon-Tilia et al., 2015). Moreover, we detected Pseudanabaena-like sequences representing 8 % of the sequence pool, consistent with previous studies (e.g., Acinas et al., 2009; Farnelid et al., 2013; Klawonn et al., 2016; Stal et al., 2003). Only 1.5 % of the sequences belonged to Aphanizomenon sp., a diazotroph known to dominate N2 fixation early in the season with salinity at 5–6 (Klawonn et al., 2016; Svedén et al., 2015). The low number found in this study might be seasonally related.
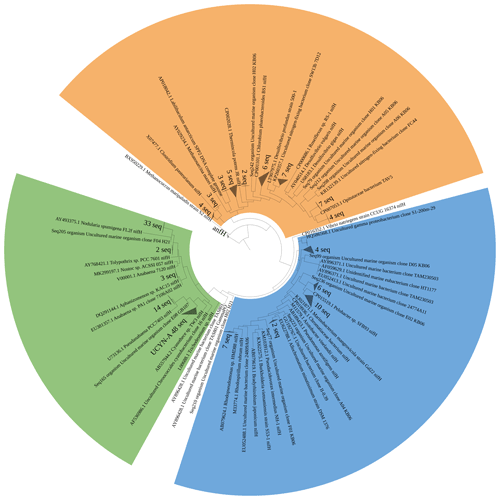
Figure 5Maximum likelihood tree of nifH amplicons (321 bp) identified by Sanger sequencing. Clusters identified are denoted as grey triangles, while identified individual sequences are denoted as Seq + NCBI submission ID. Cluster I cyanobacteria are shown in green, Cluster I Proteobacteria are shown in blue, and Cluster III diazotrophs are shown in orange.
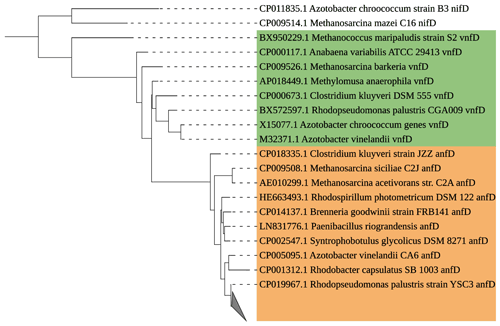
Figure 6Maximum likelihood tree of anfD/vnfD amplicons (591 bp) identified by Sanger sequencing. All recovered sequences clustered with Rhodopseudomonas palustris (grey triangle). The anfD sequences are represented in brown, and vnfD sequences are represented in green. A nifD sequence has been chosen as the outgroup.
Notably, based on our nifH sequence analysis, we did not identify Dolichospermum spp., one of the common N2-fixing cyanobacteria for the Baltic Sea (Bentzon-Tilia et al., 2015; Boström et al., 2007; Farnelid et al., 2013). However, sequences related to A. lemmermannii were recovered from our 16S rDNA amplicon sequencing (Fig. S1). This discrepancy might speak for a bias against their specific nifH genes by our approach, or the clades we found in the 16S rDNA dataset might lack a nifH gene.
We detected Nodularia-like sequences (primer and probe constructed against N. spumigena and environmental Nodularia clusters; see Boström et al., 2007) in abundances of 3.37 × 105 to 4.06 × 107 copies L−1 at 3–5 m water depth and 8.64 × 104–4.46 × 106 copies L−1 at water depths of 20–41 m. Nodularia-specific nifH transcript abundance decreased over depth from 4.20 × 105 (5 m) to 3.5 × 102 (20 m) transcripts L−1 (Fig. 7). Nodularia followed the temperature–salinity gradient, with the highest abundance (up to 1.6 × 107 copies L−1) in waters with salinity at 10 or below (the Bornholm and eastern Gotland basins) and the lowest abundances (up 1.46 × 106 copies L−1) in waters with a salinity above 10 (Kiel Fjord and the Arkona Basin). Nodularia-specific nifH gene and transcript abundances were in the same range as previously described (106 gene copies L−1 and 105 transcripts L−1; Farnelid et al., 2013). UCYN-A was identified based on both nifH and 16S rDNA sequences and quantified using cluster-specific qPCR. UCYN-A was present in abundances of 3.73 × 103–9.97 × 107 copies L−1, similar to the one available previous study wherein the abundance of nifH specific for UCYN-A peaked in September (approximately 106 copies L−1) (Bentzon-Tilia et al., 2015) (Fig. 7). The same study showed nifH expression (roughly 103 transcripts L−1) of UCYN-A in the Baltic Sea surface waters (1 m). These transcript abundances are comparable with those obtained from our study, with transcript abundances between 2.98 × 102 and 3.73 × 102 transcripts L−1 at 5 m water depth and up to 3.71 × 105 transcripts L−1 at a water depth of 20 m (Fig. 7). Notably, the abovementioned study took place in the Danish Strait and thus in more saline waters (salinity of 13–17).
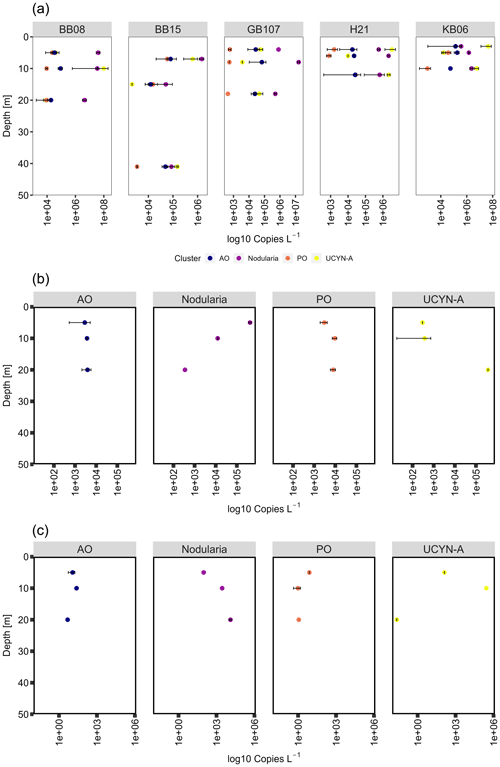
Figure 7Cluster-specific nifH (a) gene, (b) transcript abundances, and (c) transcript per gene copy of Nodularia, UCYN-A, and gamma PO/AO. In general, both cyanobacterial groups are decreasing in nifH gene abundance over depth at all stations. Transcript abundances of Nodularia–nifH were higher in surface waters (5 m), contrary to UCYN-A, which showed an opposite trend. Gamma PO/AO gene and transcript abundance generally increased over depth.
Contrary to Nodularia, UCYN-A reached the highest abundances (up to 4.52 × 107 copies L−1) in higher-salinity waters impacted by the North Sea (the Kiel Fjord and Arkona Basin) and the lowest abundance (up to 6.29 × 105 copies L−1) in low-salinity waters (Fig. 7, Supplement Fig. S1). UCYN-A is increasingly found throughout the oceans, often playing an important role in N2 fixation (e.g., Martínez-Pérez et al., 2016; Tang and Cassar, 2019). Over recent years, UCYN-A has been shown to have a crucial part in N2 fixation and has been identified all over the globe from polar to tropical regions, tolerating between 4 and 30 ∘C (Gradoville et al., 2020; Harding et al., 2018; Martínez-Pérez et al., 2016; Mills et al., 2020; Short and Zehr, 2007; Tang and Cassar, 2019). Despite UCYN-A exhibiting the highest abundances in Baltic Sea waters with higher salinity, we found UCYN-A present and active across the salinity gradient.
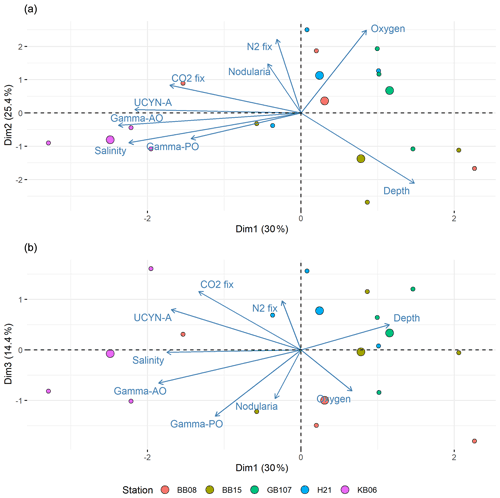
Figure 8Principal component analysis (PCA) of components 1 and 2 (a) and components 1 and 3 (b). Positive correlations were identified between salinity and UCYN-A, while Nodularia and salinity are negatively correlated. Moreover, N2 fixation correlated positively with Nodularia. Stations are color-coded. Each circle denotes one sampling point; bigger circles indicate the center for each station.
Besides those cyanobacterial diazotrophs, we detected several clades of Proteobacteria related to Rhodopseudomonas palustris (4 %), Pelobacter sp. (9 %), and Gammaproteobacteria of the gamma AO clade (3 %, Langlois et al., 2008). We quantified gammaproteobacterial nifH in abundances between 1.78 × 104 and 1.82 × 105 copies L−1 (gamma AO) and their transcripts in abundances of 2.89 × 103–3.91 × 103 transcripts L−1. Despite lack of clones clustering with gamma PO, the group could be detected in abundances between 8.21 × 102 and 1.37 × 105 copies L−1 and their transcripts in abundances between 3.04 × 103 and 8.05 × 103 transcripts L−1, indicating a potential contribution to N2 fixation of those clades (Fig. 7). Diazotrophic Proteobacteria are common in the ocean; however, their role for N2 fixation is not fully understood (Benavides et al., 2018; Chen et al., 2019; Turk-Kubo et al., 2014). Diazotrophs related to Cluster III were dominated by Desulfovibrio-like (1.5 %), Opitutaceae-like (6 %), and Clostridium-like sequences (1.5 %). However, both nifH gene and transcript abundances were below the detection limit of our qPCR. Altogether, the gene and transcript abundances of cyanobacterial diazotrophs were 3 to 4 orders of magnitude higher than those of non-cyanobacterial clusters, suggesting that heterotrophic microbes may have only played a minor role for N2 fixation in Baltic Sea surface waters during our cruise.
In the pool of alternative nitrogenase sequences of the anfD type, we identified the purple non-sulfur bacterium Rhodopseudomonas palustris. We could not recover any vnfD sequences, which might be an adaptation to low vanadium concentrations in the Baltic Sea (Bauer et al., 2017). The role of alternative nitrogenases in the environment is poorly understood, partly due to a lack of data and detection systems. Our data, however, show the presence of alternative nitrogenases in the Baltic Sea surface water, which may sustain N2 fixation under certain conditions, for example in anaerobic micro-niches (Bertagnolli and Stewart, 2018; Farnelid et al., 2019; Paerl and Prufert, 1987; Pelve et al., 2017), including sinking particles (Chakraborty et al., 2021; Pedersen et al., 2018).
3.4 Factors impacting the diazotrophic distribution
To identify parameters impacting the distribution of diazotrophs during our cruise, we carried out a simper analysis. Interestingly, pH and temperature were less important for describing the diazotrophic distribution. The PCA suggested that depth and salinity explained the distribution of both the diazotrophic community and N2 fixation best (Fig. 8). Moreover, it revealed a correlation of N2 fixation and the Nodularia abundance and less of a correlation between N2 fixation and UCYN-A or other diazotroph clusters (Fig. 8). A decreasing salinity would thus not only promote the distribution of Nodularia-like diazotrophs and decrease UCYN-A abundances, but also promote N2 fixation rates in such a future scenario. Intuitively, it is of little surprise that our data (Fig. 8) and previous studies indicate salinity to be a key control for cyanobacterial distribution and N2 fixation in the Baltic Sea (Dupont et al., 2014; Olofsson et al., 2020; Rakko and Seppälä, 2014). A recent study also suggested a positive correlation between UCYN-A and salinity (Li et al., 2021), while a study based on a compiled dataset (1979–2017) indicated that salinity does not affect the biovolumes of the filamentous N. spumigena but rather species interactions (Karlberg and Wulff, 2013; Olofsson et al., 2020). Our study cannot evaluate such interactions and their impact of N2 fixation; however, our data do point towards Nodularia expanding into low-salinity waters in the future, thus complementing UCYN-A and possibly increasing N2 fixation rates in those waters.
Our analysis did not show temperature and pH to be major descriptors of N2 fixation during this cruise, consistent with previous findings with elevated CO2 concentrations not causing any response in N2 fixation in the Baltic Sea (Olofsson et al., 2019; Paul et al., 2016; Wulff et al., 2018). Moreover, a very recent study showed that ocean acidification has an impact on the diazotroph community composition and ecology and can decrease bulk N2 fixation rates in the subtropical Atlantic Ocean (Singh et al., 2021). Most likely, the contradicting results are due to other factors obscuring the stimulation by N2 fixation (Karlberg and Wulff, 2013; Wannicke et al., 2018). Besides pH, temperature has also been suggested as a control on diazotrophy, either directly or indirectly, by impacting O2 solubility (Stal, 2009).
Also, increasing nutrient loads in the Baltic Sea might further stimulate primary production, leading to increased anoxia and PO release from sediments (Ingall and Jahnke, 1997), which will, in turn, fuel N2 fixation and primary production (Canfield, 2006; Saraiva et al., 2019). Filamentous cyanobacteria benefit from excess PO (Olofsson et al., 2016); thus, excess PO resulting from progressive deoxygenation might stimulate blooms of Nodularia (Degerholm et al., 2006; Schoffelen et al., 2018).
In the case of a potential future freshening of the upper water column (Liblik and Lips, 2019) in combination with increased PO availability through both land-derived influx and phosphorus mobilization via bottom-water anoxia (Gustafsson et al., 2017; Ingall and Jahnke, 1997; Stigebrandt and Andersson, 2020; Vahtera et al., 2007), N2 fixation by, e.g., Nodularia will likely increase in the region covered by our cruise. This could lead to a scenario with increased bloom formations and expansion of such large heterocystous cyanobacteria across the Baltic Sea, as previously observed (Kahru et al., 1994; Kahru and Elmgren, 2014). An increased organic matter load would possibly lead to enhanced respiration by heterotrophic microbes, promoting the further expansion of O2-depleted waters. This could lead to increased denitrification, resulting in N loss and emission of N2O, fueling global warming with an increase in euxinic events as already observed in coastal Baltic waters (Breitburg et al., 2018; Carstensen et al., 2014; Lennartz et al., 2014). Further, blooms of Nodularia could lead to an increased load of Baltic Sea waters with the toxin nodularin (Sivonen et al., 1989), which can harm Baltic Sea biota and poison animals (Main et al., 1977; Nehring, 1993) and ultimately humans by impacting, e.g., the fishery industry (Karjalainen et al., 2007). The latest studies, however, show that projection of increased sea level rise counteracts freshening events in the Baltic Sea, making it uncertain whether salinity will increase or decrease in the future (Meier et al., 2021, 2022). Regardless, potential changes in salinity might impact the diazotrophic community as described above. The uncertainty in changing salinity makes it unclear to what extent diazotrophs might be impacted.
During our cruise, we explored the diazotrophic community composition and N2 fixation rates along the natural salinity gradient in the southern Baltic Sea in the fall season. N2 fixation rates were detectable but low for the Baltic Sea and sustained by a diazotrophic community dominated by the heterocystous cyanobacterium Nodularia and the small unicellular cyanobacterium UCYN-A. The two different types of cyanobacteria occupied two different niches defined by salinity ranges, with Nodularia dominating in lower-salinity waters (8–9) and UCYN-A in high-salinity waters (>10). Statistical analysis revealed that N2 fixation is quantitatively mainly driven by Nodularia clades and that both Nodularia abundances and N2 fixation rates are best explained by salinity. In the context of a predicted freshening of the Baltic Sea, the habitat of Nodularia-like heterocystous cyanobacteria would extend towards the southwestern part of the Baltic Sea, possibly replacing the community of UCYN-A and increasing N2 fixation in those waters. Enhanced N2 fixation might facilitate primary productivity, and organic matter export to waters below the euphotic zone and could thus have severe impacts on the Baltic Sea biogeochemistry including increased respiration, as well as O2 and N loss.
The datasets presented in this study can be found in online repositories. Accession number(s) can be found in NCBI with MZ063808-MZ063873 and MZ063874-MZ064057 as well as 16S rDNA with accession number SAMN20309768-SAMN20309783.
The supplement related to this article is available online at: https://doi.org/10.5194/os-18-401-2022-supplement.
CFR and CRL designed the experiments and CFR carried out the experimental work. JJ and IS designed the sampling strategy during the expedition and contributed essential datasets. CFR and CRL prepared the paper with contributions from all the co-authors.
The contact author has declared that neither they nor their co-authors have any competing interests.
Publisher's note: Copernicus Publications remains neutral with regard to jurisdictional claims in published maps and institutional affiliations.
We thank the captain, crew, and chief scientist Jörg Süling of Alkor 528 for their support during the cruise. We thank Erik Laursen and Rikke Orloff Holm for their technical assistance. We thank Harri Kuosa and Malin Olofsson for reviewing the paper. Further support was received from the Nordcee labs at SDU and the GEOMAR-Helmholtz Centre for Ocean Research Kiel by providing access to their research vessel and facilities.
This research has been supported by the Villum Fonden (grant nos. 16518 to Donald Canfield and 29411 to Carolin Regina Löscher) and the European Union Horizon 2020 (GoJelly (grant no. 774499 to Jamileh Javidpour)).
This paper was edited by Markus Meier and reviewed by Malin Olofsson and Harri Kuosa.
Acinas, S. G., Haverkamp, T. H. A., Huisman, J., and Stal, L. J.: Phenotypic and genetic diversification of Pseudanabaena spp. (cyanobacteria), ISME J., 3, 31–46, https://doi.org/10.1038/ismej.2008.78, 2009.
Affourtit, J., Zehr, J. P., and Paerl, H. W.: Distribution of nitrogen-fixing microorganisms along the Neuse River Estuary, North Carolina, Microb. Ecol., 41, 114–123, https://doi.org/10.1007/s002480000090, 2001.
Anbar, A. D.: Oceans: Elements and evolution, Science, 322, 1481–1483, https://doi.org/10.1126/science.1163100, 2008.
Arrigo, K. R.: Marine microorganisms and global nutrient cycles, Nature, 438, 7064, https://doi.org/10.1038/nature04265, 2005.
Bauer, S., Blomqvist, S., and Ingri, J.: Distribution of dissolved and suspended particulate molybdenum, vanadium, and tungsten in the Baltic Sea, Mar. Chem., 196, 135–147, https://doi.org/10.1016/j.marchem.2017.08.010, 2017.
Bellenger, J. P., Wichard, T., Xu, Y., and Kraepiel, A. M. L.: Essential metals for nitrogen fixation in a free-living N2-fixing bacterium: Chelation, homeostasis and high use efficiency, Environ. Microbiol., 13, 1395–1411, https://doi.org/10.1111/j.1462-2920.2011.02440.x, 2011.
Bellenger, J. P., Xu, Y., Zhang, X., Morel, F. M. M., and Kraepiel, A. M. L.: Possible contribution of alternative nitrogenases to nitrogen fixation by asymbiotic N2-fixing bacteria in soils, Soil Biol. Biochem., 69, 413–420, https://doi.org/10.1016/j.soilbio.2013.11.015, 2014.
Benavides, M., Martias, C., Elifantz, H., Berman-Frank, I., Dupouy, C., and Bonnet, S.: Dissolved organic matter influences N2 fixation in the New Caledonian lagoon (western tropical South Pacific), Front. Mar. Sci., 5, 1–12, https://doi.org/10.3389/fmars.2018.00089, 2018.
Bennett, W. W. and Canfield, D. E.: Redox-sensitive trace metals as paleoredox proxies: A review and analysis of data from modern sediments, Earth Sci. Rev., 204, 103175, https://doi.org/10.1016/j.earscirev.2020.103175, 2020.
Bentzon-Tilia, M., Traving, S. J., Mantikci, M., Knudsen-Leerbeck, H., Hansen, J. L. S., Markager, S., and Riemann, L.: Significant N 2 fixation by heterotrophs, photoheterotrophs and heterocystous cyanobacteria in two temperate estuaries, ISME J., 9, 273–285, https://doi.org/10.1038/ismej.2014.119, 2015.
Bertagnolli, A. D. and Stewart, F. J.: Microbial niches in marine oxygen minimum zones, Nat. Rev. Microbiol., 16, 723–729, https://doi.org/10.1038/s41579-018-0087-z, 2018.
Bertine, K. K. and Turekian, K. K.: Molybdenum in marine deposits, Geochim. Cosmochim. Ac., 37, 1415–1434, https://doi.org/10.1016/0016-7037(73)90080-X, 1973.
Betancourt, D. A., Loveless, T. M., Brown, J. W., and Bishop, P. E.: Characterization of diazotrophs containing Mo-independent nitrogenases, isolated from diverse natural environments, Appl. Environ. Microbiol., 74, 3471–3480, https://doi.org/10.1128/AEM.02694-07, 2008.
Boström, K. H., Riemann, L., Zweifel, U. L., and Hagström, Å.: Nodularia sp. nifH gene transcripts in the Baltic Sea proper, J. Plankton Res., 29, 391–399, https://doi.org/10.1093/plankt/fbm019, 2007.
Brauer, V. S., Stomp, M., Rosso, C., Van Beusekom, S. A. M., Emmerich, B., Stal, L. J., and Huisman, J.: Low temperature delays timing and enhances the cost of nitrogen fixation in the unicellular cyanobacterium Cyanothece, ISME J., 7, 2105–2115, https://doi.org/10.1038/ismej.2013.103, 2013.
Breitburg, D., Levin, L. A., Oschlies, A., Grégoire, M., Chavez, F. P., Conley, D. J., Garçon, V., Gilbert, D., Gutiérrez, D., Isensee, K., Jacinto, G. S., Limburg, K. E., Montes, I., Naqvi, S. W. A., Pitcher, G. C., Rabalais, N. N., Roman, M. R., Rose, K. A., Seibel, B. A., Telszewski, M., Yasuhara, M., and Zhang, J.: Declining oxygen in the global ocean and coastal waters, Science, 359, 6371, https://doi.org/10.1126/science.aam7240, 2018.
Callahan, B. J., McMurdie, P. J., Rosen, M. J., Han, A. W., Johnson, A. J. A., and Holmes, S. P.: DADA2: High-resolution sample inference from Illumina amplicon data, Nat. Methods, 13, 581–583, https://doi.org/10.1038/nmeth.3869, 2016.
Canfield, D. E.: Models of oxic respiration, denitrification and sulfate reduction in zones of coastal upwelling, Geochim. Cosmochim. Ac., 70, 5753–5765, https://doi.org/10.1016/j.gca.2006.07.023, 2006.
Carpenter, E. J. and Capone, D. G.: Nitrogen fixation in the marine environment, in: Nitrogen in marine environment, chap. 4, 2nd Edn., Elsevier Science and Technology, 141–198, https://doi.org/10.1016/B978-0-12-372522-6.00004-9, 2008.
Carstensen, J., Andersen, J. H., Gustafsson, B. G., and Conley, D. J.: Deoxygenation of the baltic sea during the last century, P. Natl. Acad. Sci. USA, 111, 5628–5633, https://doi.org/10.1073/pnas.1323156111, 2014.
Chakraborty, S., Andersen, K. H., Follows, M. J., and Riemann, L.: Quantifying nitrogen fixation by heterotrophic bacteria in sinking marine particles, Nat. Commun., 12, 4085, https://doi.org/10.1038/s41467-021-23875-6, 2021.
Chen, M., Lu, Y., Jiao, N., Tian, J., Kao, S. J., and Zhang, Y.: Biogeographic drivers of diazotrophs in the western Pacific Ocean, Limnol. Oceanogr., 64, 1403–1421, https://doi.org/10.1002/lno.11123, 2019.
Chisnell, J. R., Premakumar, R., and Bishop, P. E.: Purification of a second alternative nitrogenase from a nifHDK deletion strain of Azotobacter vinelandii., J. Bacteriol., 170, 27–33, https://doi.org/10.1128/jb.170.1.27-33.1988, 1988.
Christiansen, C. F. and Löscher, C. R.: Facets of diazotrophy in the OMZ off Peru revisited- what we couldn't see from a single marker gene approach, BioarXiv [preprint], https://doi.org/10.1101/558072, 2019.
Church, M. J., Mahaffey, C., Letelier, R. M., Lukas, R., Zehr, J. P., and Karl, D. M.: Physical forcing of nitrogen fixation and diazotroph community structure in the North Pacific subtropical gyre, Global Biogeochem. Cy., 23, 2, https://doi.org/10.1029/2008GB003418, 2009.
Conley, D. J., Humborg, C., Rahm, L., Savchuk, O. P., and Wulff, F.: Hypoxia in the baltic sea and basin-scale changes in phosphorus biogeochemistry, Environ. Sci. Technol., 36, 5315–5320, https://doi.org/10.1021/es025763w, 2002.
Crusius, J., Calvert, S., Pedersen, T., and Sage, D.: Rhenium and molybdenum enrichments in sediments as indicators of oxic, suboxic and sulfidic conditions of deposition, Earth Planet. Sc. Lett., 145, 65–78, https://doi.org/10.1016/s0012-821x(96)00204-x, 1996.
Dähnke, K. and Thamdrup, B.: Nitrogen isotope dynamics and fractionation during sedimentary denitrification in Boknis Eck, Baltic Sea, Biogeosciences, 10, 3079–3088, https://doi.org/10.5194/bg-10-3079-2013, 2013.
Degerholm, J., Gundersen, K., Bergman, B., and Söderbäck, E.: Phosphorus-limited growth dynamics in two Baltic Sea cyanobacteria, Nodularia sp. and Aphanizomenon sp., FEMS Microbiol. Ecol., 58, 323–332, https://doi.org/10.1111/j.1574-6941.2006.00180.x, 2006.
Delwiche, C. C. and Steyn, P. L.: Nitrogen Isotope Fractionation in Soils and Microbial Reactions, Environ. Sci. Technol., 4, 929–935, https://doi.org/10.1021/es60046a004, 1970.
Dera, J. and Woźniak, B.: Solar radiation in the baltic sea, Oceanologia, 52, 533–582, https://doi.org/10.5697/oc.52-4.533, 2010.
Diaz, R. J. and Rosenberg, R.: Spreading Dead Zones and Consequences for Marine Ecosystems, Am. Assoc. Adv. Sci., 321, 926–929, 2008.
Dicker, H. J. and Smith, D. W.: Effects of Salinity on Acetylene Reduction (Nitrogen Fixation) and Respiration in a Marine Azotobacter , Appl. Environ. Microbiol., 42, 740–744, https://doi.org/10.1128/aem.42.4.740-744.1981, 1981.
Dixon, R. and Kahn, D.: Genetic regulation of biological nitrogen fixation, Nat. Rev. Microbiol., 2, 621–631, https://doi.org/10.1038/nrmicro954, 2004.
Dore, J. E., Brum, J. R., Tupas, L. M., and Karl, D. M.: Seasonal and interannual variability in sources of nitrogen supporting export in the oligotrophic subtropical North Pacific Ocean, Limnol. Oceanogr., 47, 1595–1607, https://doi.org/10.4319/lo.2002.47.6.1595, 2002.
Dupont, C. L., Larsson, J., Yooseph, S., Ininbergs, K., Goll, J., Asplund-Samuelsson, J., McCrow, J. P., Celepli, N., Allen, L. Z., Ekman, M., Lucas, A. J., Hagström, Å., Thiagarajan, M., Brindefalk, B., Richter, A. R., Andersson, A. F., Tenney, A., Lundin, D., Tovchigrechko, A., Nylander, J. A. A., Brami, D., Badger, J. H., Allen, A. E., Rusch, D. B., Hoffman, J., Norrby, E., Friedman, R., Pinhassi, J., Venter, J. C., and Bergman, B.: Functional tradeoffs underpin salinity-driven divergence in microbial community composition, PLoS One, 9, e89549, https://doi.org/10.1371/journal.pone.0089549, 2014.
Englund, B. and Meyerson, H.: In situ Measurement of Nitrogen Fixation at Low Temperatures, Wiley, 25, 283–287, 1974.
Farnelid, H., Öberg, T., and Riemann, L.: Identity and dynamics of putative N2-fixing picoplankton in the Baltic Sea proper suggest complex patterns of regulation, Environ. Microbiol. Rep., 1, 145–154, https://doi.org/10.1111/j.1758-2229.2009.00021.x, 2009.
Farnelid, H., Bentzon-Tilia, M., Andersson, A. F., Bertilsson, S., Jost, G., Labrenz, M., Jürgens, K., and Riemann, L.: Active nitrogen-fixing heterotrophic bacteria at and below the chemocline of the central Baltic Sea, ISME J., 7, 1413–1423, https://doi.org/10.1038/ismej.2013.26, 2013.
Farnelid, H., Turk-Kubo, K., Ploug, H., Ossolinski, J. E., Collins, J. R., Van Mooy, B. A. S., and Zehr, J. P.: Diverse diazotrophs are present on sinking particles in the North Pacific Subtropical Gyre, ISME J., 13, 170–182, https://doi.org/10.1038/s41396-018-0259-x, 2019.
Fernandez, C., Farías, L., and Ulloa, O.: Nitrogen fixation in denitrified marine waters, PLoS One, 6, e20539, https://doi.org/10.1371/journal.pone.0020539, 2011.
Fonseca-Batista, D., Li, X., Riou, V., Michotey, V., Deman, F., Fripiat, F., Guasco, S., Brion, N., Lemaitre, N., Tonnard, M., Gallinari, M., Planquette, H., Planchon, F., Sarthou, G., Elskens, M., Laroche, J., Chou, L., and Dehairs, F.: Evidence of high N2 fixation rates in the temperate northeast Atlantic, Biogeosciences, 16, 999–1017, https://doi.org/10.5194/bg-16-999-2019, 2019.
Gradoville, M. R., Farnelid, H., White, A. E., Turk-Kubo, K. A., Stewart, B., Ribalet, F., Ferrón, S., Pinedo-gonzalez, P., Armbrust, E. V., Karl, D. M., John, S., and Zehr, J. P.: Latitudinal constraints on the abundance and activity of the cyanobacterium UCYN-A and other marine diazotrophs in the North Pacific, Limnol. Oceanogr., 9999, 1–18, https://doi.org/10.1002/lno.11423, 2020.
Grasshoff, G., Kremling, K., and Erhardt, M.: Methods of seawater analysis, Wiley VCH, Weinheim, 3rd Edn., ISBN 3527295895, 1999.
Gruber, N.: A bigger nitrogen fix, Nature, 436, 786–787, https://doi.org/10.1038/436786a, 2005.
Gustafsson, E., Savchuk, O. P., Gustafsson, B. G., and Müller-Karulis, B.: Key processes in the coupled carbon, nitrogen, and phosphorus cycling of the Baltic Sea, Biogeochemistry, 134, 301–317, https://doi.org/10.1007/s10533-017-0361-6, 2017.
Hall, P. 0. J. and Aller, R. C.: Rapid, small-volume, flow injection analysis for SCO2, and NH in marine and freshwaters, Limnol. Oceanogr., 37, 1113–1119, https://doi.org/10.4319/lo.1992.37.5.1113, 1992.
Hall, T. A.: BioEdit: a user-friendly biological sequence alignment editor and analysis program for Windows, https://doi.org/10.14601/Phytopathol_Mediterr-14998u1.29, 1999.
Hamersley, M. R., Turk, K. A., Leinweber, A., Gruber, N., Zehr, J. P., Gunderson, T., and Capone, D. G.: Nitrogen fixation within the water column associated with two hypoxic basins in the Southern California Bight, Aquat. Microb. Ecol., 63, 193–205, https://doi.org/10.3354/ame01494, 2011.
Harding, K., Turk-Kubo, K. A., Sipler, R. E., Mills, M. M., Bronk, D. A., and Zehr, J. P.: Symbiotic unicellular cyanobacteria fix nitrogen in the Arctic Ocean, P. Natl. Acad. Sci. USA, 115, 13371–13375, https://doi.org/10.1073/pnas.1813658115, 2018.
Helcom: HELCOM, https://helcom.fi/baltic-sea-trends/indicators/ (last access: 9 June 2021), 2018.
Hietanen, S., Jäntti, H., Buizert, C., Jürgens, K., Labrenz, M., Voss, M., and Kuparinen, J.: Hypoxia and nitrogen processing in the Baltic Sea water column, Limnol. Oceanogr., 57, 325–337, https://doi.org/10.4319/lo.2012.57.1.0325, 2012.
ICES Copenhagen: International Council for the Exploration of the Sea (ICES) – Dataset on Ocean Hydrography, https://ocean.ices.dk/ (last access: 9 June 2021), 2020.
Ingall, E. and Jahnke, R.: Influence of water-column anoxia on the elemental fractionation of carbon and phosphorus during sediment diagenesis, Mar. Geol., 139, 219–229, https://doi.org/10.1016/S0025-3227(96)00112-0, 1997.
Janson, S., Carpenter, E. J., and Bergman, B.: Fine structure and immunolocalisation of proteins in Aphanizomenon sp. from the Baltic Sea, J. Europ. J. Phycol., 29, 203–211, https://doi.org/10.1080/09670269400650651, 1994.
Jayakumar, A., Al-Rshaidat, M. M. D., Ward, B. B., and Mulholland, M. R.: Diversity, distribution, and expression of diazotroph nifH genes in oxygen-deficient waters of the Arabian Sea, FEMS Microbiol. Ecol., 82, 597–606, https://doi.org/10.1111/j.1574-6941.2012.01430.x, 2012.
Jayakumar, A., Chang, B. X., Widner, B., Bernhardt, P., Mulholland, M. R., and Ward, B. B.: Biological nitrogen fixation in the oxygen-minimum region of the eastern tropical North Pacific ocean, ISME J., 11, 2356–2367, https://doi.org/10.1038/ismej.2017.97, 2017.
Kahru, M. and Elmgren, R.: Multidecadal time series of satellite-detected accumulations of cyanobacteria in the Baltic Sea, Biogeosciences, 11, 3619–3633, https://doi.org/10.5194/bg-11-3619-2014, 2014.
Kahru, M., Horstmann, U., and Rud, O.: Satellite detection of increased cyanobacteria blooms in the Baltic Sea: Natural fluctuation or ecosystem change?, Ambio, 23, 469–472, 1994.
Karjalainen, M., Engström-Öst, J., Korpinen, S., Peltonen, H., Pääkkönen, J. P., Rönkkönen, S., Suikkanen, S., and Viitasalo, M.: Ecosystem consequences of cyanobacteria in the northern Baltic Sea, Ambio, 36, 195–202, https://doi.org/10.1579/0044-7447(2007)36[195:ECOCIT]2.0.CO;2, 2007.
Karlberg, M. and Wulff, A.: Impact of temperature and species interaction on filamentous cyanobacteria may be more important than salinity and increased pCO2 levels, Mar. Biol., 160, 2063–2072, https://doi.org/10.1007/s00227-012-2078-3, 2013.
Kassambara, A. and Mundt, F.: factoextra: Extract and Visualize the Results of Multivariate Data Analyses, https://cran.r-project.org/web/packages/factoextra/index.html, 2020.
Keeling, R. F., Körtzinger, A., and Gruber, N.: Ocean Deoxygenation in a Warming World, Ann. Rev. Mar. Sci., 2, 199–229, https://doi.org/10.1146/annurev.marine.010908.163855, 2010.
Klawonn, I., Nahar, N., Walve, J., Andersson, B., Olofsson, M., Svedén, J. B., Littmann, S., Whitehouse, M. J., Kuypers, M. M. M., and Ploug, H.: Cell-specific nitrogen- and carbon-fixation of cyanobacteria in a temperate marine system (Baltic Sea), Environ. Microbiol., 18, 4596–4609, https://doi.org/10.1111/1462-2920.13557, 2016.
Kumar, S., Stecher, G., Li, M., Knyaz, C., and Tamura, K.: MEGA X: Molecular evolutionary genetics analysis across computing platforms, Mol. Biol. Evol., 35, 1547–1549, https://doi.org/10.1093/molbev/msy096, 2018.
Langlois, R. J., Hümmer, D., and LaRoche, J.: Abundances and distributions of the dominant nifH phylotypes in the Northern Atlantic Ocean, Appl. Environ. Microbiol., 74, 1922–1931, https://doi.org/10.1128/AEM.01720-07, 2008.
Larsson, U., Hajdu, S., Walve, J., and Elmgren, R.: Baltic Sea Nitrogen Fixation Estimated from the Summer Increase in Upper Mixed Layer, Limno. Oceanogr., 46, 811–820, 2001.
Lehtimäki, J., Moisander, P., Sivonen, K., and Kononen, K.: Growth, nitrogen fixation, and Nodularin production by two Baltic Sea cyanobacteria, Appl. Environ. Microbiol., 63, 1647–1656, https://doi.org/10.1128/aem.63.5.1647-1656.1997, 1997.
Lennartz, S. T., Lehmann, A., Herrford, J., Malien, F., Hansen, H. P., Biester, H., and Bange, H. W.: Long-term trends at the Boknis Eck time series station (Baltic Sea), 1957–2013: Does climate change counteract the decline in eutrophication?, Biogeosciences, 11, 6323–6339, https://doi.org/10.5194/bg-11-6323-2014, 2014.
Leppanen, J., Niemi, A., and Rinne, I.: Nitrogen fixation of Cyanobacteria (blue-green algae) and the nitrogen cycle of the Baltic Sea, Symbiosis, 6, 181–194, 1988.
Li, L., Wu, C., Huang, D., Ding, C., Wei, Y., and Sun, J.: Integrating Stochastic and Deterministic Process in the Biogeography of N2-Fixing Cyanobacterium Candidatus Atelocyanobacterium Thalassa, Front. Microbiol., 12, 1–15, https://doi.org/10.3389/fmicb.2021.654646, 2021.
Liblik, T. and Lips, U.: Stratification has strengthened in the baltic sea – an analysis of 35 years of observational data, Front. Earth Sci., 7, 1–15, https://doi.org/10.3389/feart.2019.00174, 2019.
Löscher, C. R., Großkopf, T., Desai, F. D., Gill, D., Schunck, H., Croot, P. L., Schlosser, C., Neulinger, S. C., Pinnow, N., Lavik, G., Kuypers, M. M. M., Laroche, J., and Schmitz, R. A.: Facets of diazotrophy in the oxygen minimum zone waters off Peru, ISME J., 8, 1–13, https://doi.org/10.1038/ismej.2014.71, 2014.
Loveless, T. M., Saah, J. R., and Bishop, P. E.: Isolation of nitrogen-fixing bacteria containing molybdenum-independent nitrogenases from natural environments, Appl. Environ. Microbiol., 65, 4223–4226, https://doi.org/10.1128/aem.65.9.4223-4226.1999, 1999.
Maar, M., Markager, S., Madsen, K. S., Windolf, J., Lyngsgaard, M. M., Andersen, H. E., and Møller, E. F.: The importance of local versus external nutrient loads for Chl a and primary production in the Western Baltic Sea, Ecol. Modell., 320, 258–272, https://doi.org/10.1016/j.ecolmodel.2015.09.023, 2016.
Main, D. C., Berry, P. H., Peet, R. L., and Robertson, J. P.: Sheep mortalities associated with the blue green alga Nodularia spumigena, Austr. Vet. J., 53, 578–581, https://doi.org/10.1111/j.1751-0813.1977.tb15830.x, 1977.
Man-Aharonovich, D., Kress, N., Zeev, E. B., Berman-Frank, I., and Béjà, O.: Molecular ecology of nifH genes and transcripts in the eastern Mediterranean Sea, Environ. Microbiol., 9, 2354–2363, https://doi.org/10.1111/j.1462-2920.2007.01353.x, 2007.
Marino, R., Chan, F., Howarth, R. W., Pace, M. L., and Likens, G. E.: Ecological constraints on planktonic nitrogen fixation in saline estuaries. I. Nutrient and trophic controls, Mar. Ecol. Prog. Ser., 309, 25–39, https://doi.org/10.3354/meps309025, 2006.
Martínez-Pérez, C., Mohr, W.,; Löscher, C. R., Dekaezemacker, J., Littmann, S., Yilmaz, P., Lehnen, N., Fuchs, B. M., Lavik, G., Schmitz, R. A., LaRoche, J., and Kuypers, M. M. M: The small unicellular diazotrophic symbiont, UCYN-A, is a key player in the marine nitrogen cycle, Nat. Microbiol., 1, 16163, https://doi.org/10.1038/nmicrobiol.2016.163, 2016.
McMurdie, P. J. and Holmes, S.: Phyloseq: An R Package for Reproducible Interactive Analysis and Graphics of Microbiome Census Data, PLoS One, 8, e61217, https://doi.org/10.1371/journal.pone.0061217, 2013.
McRose, D. L., Zhang, X., Kraepiel, A. M. L., and Morel, F. M. M.: Diversity and activity of alternative nitrogenases in sequenced genomes and coastal environments, Front. Microbiol., 8, 1–13, https://doi.org/10.3389/fmicb.2017.00267, 2017.
Meier, H. E. M., Dieterich, C., and Gröger, M.: Natural variability is a large source of uncertainty in future projections of hypoxia in the Baltic Sea, Commun. Earth Environ., 2, 50, https://doi.org/10.1038/s43247-021-00115-9, 2021.
Meier, H. E. M., Dieterich, C., Gröger, M., Dutheil, C., Börgel, F., Safonova, K., Christensen, O. B., and Kjellström, E.: Oceanographic regional climate projections for the Baltic Sea until 2100, Earth Syst. Dynam., 13, 159–199, https://doi.org/10.5194/esd-13-159-2022, 2022.
Mills, M. M., Turk-Kubo, K. A., van Dijken, G. L., Henke, B. A., Harding, K., Wilson, S. T., Arrigo, K. R., and Zehr, J. P.: Unusual marine cyanobacteria/haptophyte symbiosis relies on N2 fixation even in N-rich environments, ISME J., 14, 2395–2406, https://doi.org/10.1038/s41396-020-0691-6, 2020.
Moisander, P. H., Beinart, R. A., Hewson, I., White, A. E., Johnson, K. S., Carlson, C. A., Montoya, J. P., Zehr, J. P., Moisander, P. H., Beinart, R. A., White, A. E., Johnson, K. S., Carlson, C. A., Montoya, J. P., and Zehr, J. P.: Unicellular Cyanobacterial Distributions Broaden the Oceanic N2 Fixation Domain, Am. Assoc. Adv. Sci., 327, 1512–1514, 2010.
Montoya, J. P., Voss, M., Ka, P., and Capone, D. G.: A Simple, High-Precision, High-Sensitivity Tracer Assay for N2 Fixation, Appl. Environ. Microbiol., 62, 986–993, 1996.
Morford, J. L. and Emerson, S.: The geochemistry of redox sensitive trace metals in sediments, Geochim. Cosmochim. Ac., 63, 1735–1750, https://doi.org/10.1016/S0016-7037(99)00126-X, 1999.
Mus, F., Colman, D. R., Peters, J. W., and Boyd, E. S.: Geobiological feedbacks, oxygen, and the evolution of nitrogenase, Free Radic. Biol. Med., 140, 250–259, https://doi.org/10.1016/j.freeradbiomed.2019.01.050, 2019.
Nehring, S.: Mortality of dogs associated with a mass development of Nodularia spumigena (Cyanophyceae) in a brackish lake at the German North Sea coast, J. Plankton Res., 15, 867–872, https://doi.org/10.1093/plankt/15.7.867, 1993.
Oksanen, F. J., Blanchet, G., Friendly, M., Roeland Kindt, P., Legendre, D., McGlinn, P. R., Minchin, R. B. O., Simpson, G. L., Peter Solymos, M., Stevens, H. H., Szoecs, E., and Wagner, H.: vegan: Community Ecology Package, version 2.5.7, http://cran.r-project.org/, http://vegan.r-forge.r-project.org/, 2020.
Olofsson, M., Egardt, J., Singh, A., and Ploug, H.: Inorganic phosphorus enrichments in Baltic Sea water have large effects on growth, carbon fixation, and N2 fixation by Nodularia spumigena, Aquat. Microb. Ecol., 77, 111–123, https://doi.org/10.3354/ame01795, 2016.
Olofsson, M., Torstensson, A., Karlberg, M., Steinhoff, F. S., Dinasquet, J., Riemann, L., Chierici, M., and Wulff, A.: Limited response of a spring bloom community inoculated with filamentous cyanobacteria to elevated temperature and pCO2, Bot. Mar., 62, 3–16, https://doi.org/10.1515/bot-2018-0005, 2019.
Olofsson, M., Suikkanen, S., Kobos, J., Wasmund, N., and Karlson, B.: Basin-specific changes in filamentous cyanobacteria community composition across four decades in the Baltic Sea, Harmful Algae, 91, 101685, https://doi.org/10.1016/j.hal.2019.101685, 2020.
Paerl, H. W. and Prufert, L. E.: Oxygen-Poor Microzones as Potential Sites of Microbial N2 Fixation in Nitrogen-Depleted Aerobic Marine Waters, Appl. Environ. Microbiol., 53, 1078–1087, https://doi.org/10.1128/aem.53.5.1078-1087.1987, 1987.
Paul, A. J., Achterberg, E. P., Bach, L. T., Boxhammer, T., Czerny, J., Haunost, M., Schulz, K. G., Stuhr, A., and Riebesell, U.: No observed effect of ocean acidification on nitrogen biogeochemistry in a summer Baltic Sea plankton community, Biogeosciences, 13, 3901–3913, https://doi.org/10.5194/bg-13-3901-2016, 2016.
Pedersen, J. N., Bombar, D., Paerl, R. W., and Riemann, L.: Diazotrophs and N2-Fixation associated with particles in coastal estuarine waters, Front. Microbiol., 9, 1–11, https://doi.org/10.3389/fmicb.2018.02759, 2018.
Pelve, E. A., Fontanez, K. M., and DeLong, E. F.: Bacterial succession on sinking particles in the ocean's interior, Front. Microbiol., 8, 1–15, https://doi.org/10.3389/fmicb.2017.02269, 2017.
Ploug, H., Musat, N., Adam, B., Moraru, C. L., Lavik, G., Vagner, T., Bergman, B., and Kuypers, M. M. M.: Carbon and nitrogen fluxes associated with the cyanobacterium Aphanizomenon sp. in the Baltic Sea, ISME J., 4, 1215–1223, https://doi.org/10.1038/ismej.2010.53, 2010.
Ploug, H., Adam, B., Musat, N., Kalvelage, T., Lavik, G., Wolf-Gladrow, D., and Kuypers, M. M. M.: Carbon, nitrogen and O2 fluxes associated with the cyanobacterium Nodularia spumigena in the Baltic Sea, ISME J., 5, 1549–1558, https://doi.org/10.1038/ismej.2011.20, 2011.
Quast, C., Pruesse, E., Yilmaz, P., Gerken, J., Schweer, T., Yarza, P., Peplies, J., and Glöckner, F. O.: The SILVA ribosomal RNA gene database project: Improved data processing and web-based tools, Nucleic Acids Res., 41, 590–596, https://doi.org/10.1093/nar/gks1219, 2013.
R Foundation for Statistical Computing: R: A language and environment for statistical computing, version 4.0.5, https://www.r-project.org/, 2017.
Rahav, E., Herut, B., Levi, A., Mulholland, M. R., and Berman-Frank, I.: Springtime contribution of dinitrogen fixation to primary production across the Mediterranean Sea, Ocean Sci., 9, 489–498, https://doi.org/10.5194/os-9-489-2013, 2013.
Rakko, A. and Seppälä, J.: Effect of salinity on the growth rate and nutrient stoichiometry of two Baltic Sea filamentous cyanobacterial species, Est. J. Ecol., 63, 55–70, https://doi.org/10.3176/eco.2014.2.01, 2014.
Redfield, A. C.: On the proportions of Organic derivations in sea water and their relation to the composition of plankton, Univ. Press Liverpool, James Johnstone Meml., 176–192, https://hahana.soest.hawaii.edu/cmoreserver/summercourse/2012/documents/bronk_05-30-12/Redfield_1934.pdf (last access: 23 August 2021), 1934.
Reusch, T. B. H., Dierking, J., Andersson, H. C., Bonsdorff, E., Carstensen, J., Casini, M., Czajkowski, M., Hasler, B., Hinsby, K., Hyytiäinen, K., Johannesson, K., Jomaa, S., Jormalainen, V., Kuosa, H., Kurland, S., Laikre, L., Mackenzie, B. R., Margonski, P., Melzner, F., Oesterwind, D., Ojaveer, H., Refsgaard, J. C., Sandström, A., Schwarz, G., Tonderski, K., Winder, M., and Zandersen, M.: The Baltic Sea as a time machine for the future coastal ocean, Sci. Adv., 4, 5, https://doi.org/10.1126/sciadv.aar8195, 2018.
Robson, R. L., Eady, R. R., Richardson, T. H., Miller, R. W., Hawkins, M., and Postgate, J. R.: The alternative nitrogenase of Azotobacter chroococcum is a vanadium enzyme, Nature, 322, 388–390, https://doi.org/10.1038/322388a0, 1986.
Rutgersson, A., Jaagus, J., Schenk, F., and Stendel, M.: Observed changes and variability of atmospheric parameters in the Baltic Sea region during the last 200 years, Clim. Res., 61, 177–190, https://doi.org/10.3354/cr01244, 2014.
Saito, M. A., Tyler, G. J., and Ritt, J. T.: Some Thoughts on the Concept of Colimitation: Three Definitions and the Importance of Bioavailability, Limnol. Oceanogr., 53, 276–290, 2008.
Saraiva, S., Markus Meier, H. E., Andersson, H., Höglund, A., Dieterich, C., Gröger, M., Hordoir, R., and Eilola, K.: Baltic Sea ecosystem response to various nutrient load scenarios in present and future climates, Clim. Dyn., 52, 3369–3387, https://doi.org/10.1007/s00382-018-4330-0, 2019.
Saxena, H., Sahoo, D., Khan, M. A., Kumar, S., Sudheer, A. K., and Singh, A.: Dinitrogen fixation rates in the Bay of Bengal during summer monsoon, Environ. Res. Commun., 2, 051007, https://doi.org/10.1088/2515-7620/ab89fa, 2020.
Schmidtko, S., Stramma, L., and Visbeck, M.: Decline in global oceanic oxygen content during the past five decades, Nature, 542, 335–339, https://doi.org/10.1038/nature21399, 2017.
Schoffelen, N. J., Mohr, W., Ferdelman, T. G., Littmann, S., Duerschlag, J., Zubkov, M. V., Ploug, H., and Kuypers, M. M. M.: Single-cell imaging of phosphorus uptake shows that key harmful algae rely on different phosphorus sources for growth, Sci. Rep., 8, 1–14, https://doi.org/10.1038/s41598-018-35310-w, 2018.
Severin, I., Confurius-Guns, V., and Stal, L. J.: Effect of salinity on nitrogenase activity and composition of the active diazotrophic community in intertidal microbial mats, Arch. Microbiol., 194, 483–491, https://doi.org/10.1007/s00203-011-0787-5, 2012.
Short, S. M. and Zehr, J. P.: Nitrogenase gene expression in the Chesapeake Bay Estuary, Environ. Microbiol., 9, 1591–1596, https://doi.org/10.1111/j.1462-2920.2007.01258.x, 2007.
Singh, A., Bach, L. T., Löscher, C. R., Paul, A. J., Ojha, N., and Riebesell, U.: Impact of increasing carbon dioxide on dinitrogen and carbon fixation rates under oligotrophic conditions and simulated upwelling, Limnol. Oceanogr., 9999, 1–13, https://doi.org/10.1002/lno.11795, 2021.
Sivonen, K., Kononen, K., Carmichael, W. W., Dahlem, A. M., Rinehart, K. L., Kiviranta, J., and Niemela, S. I.: Occurrence of the hepatotoxic cyanobacterium Nodularia spumigena in the Baltic Sea and structure of the toxin., Appl. Environ. Microbiol., 55, 1990–1995, https://doi.org/10.1128/aem.55.8.1990-1995.1989, 1989.
Staal, M., Te Lintel Hekkert, S., Herman, P., and Stal, L. J.: Comparison of models describing light dependence of N2 fixation in heterocystous cyanobacteria, Appl. Environ. Microbiol., 68, 4679–4683, https://doi.org/10.1128/AEM.68.9.4679-4683.2002, 2002.
Stal, L. J.: Is the distribution of nitrogen-fixing cyanobacteria in the oceans related to temperature?: Minireview, Environ. Microbiol., 11, 1632–1645, https://doi.org/10.1111/j.1758-2229.2009.00016.x, 2009.
Stal, L. J., Albertano, P., Bergman, B., Von Bröckel, K., Gallon, J. R., Hayes, P. K., Sivonen, K., and Walsby, A. E.: BASIC: Baltic Sea cyanobacteria, An investigation of the structure and dynamics of water blooms of cyanobacteria in the Baltic Sea – Responses to a changing environment, Cont. Shelf Res., 23, 1695–1714, https://doi.org/10.1016/j.csr.2003.06.001, 2003.
Steward, G. F., Jenkins, B. D., Ward, B. B., Zehr, J. P., and Short, S. M.: Fingerprinting diazotroph communities in the Chesapeake Bay by using a DNA macroarray, Appl. Environ. Microbiol., 70, 1767, https://doi.org/10.1128/AEM.70.3.1767-1776.2004, 2004.
Stigebrandt, A. and Andersson, A.: The Eutrophication of the Baltic Sea has been Boosted and Perpetuated by a Major Internal Phosphorus Source, Front. Mar. Sci., 7, 572994, https://doi.org/10.3389/fmars.2020.572994, 2020.
Stramma, L., Johnson, G. C., Sprintall, J., and Mohrholz, V.: Expanding Oxygen-Minimum Zones in the Tropical Oceans, Source Sci. New Ser., 320, 655–658, 2008.
Suikkanen, S., Kaartokallio, H., Hällfors, S., Huttunen, M., and Laamanen, M.: Life cycle strategies of bloom-forming, filamentous cyanobacteria in the Baltic Sea, Deep-Sea Res. Pt. II, 57, 199–209, https://doi.org/10.1016/j.dsr2.2009.09.014, 2010.
Svedén, J. B., Adam, B., Walve, J., Nahar, N., Musat, N., Lavik, G., Whitehouse, M. J., Kuypers, M. M. M., and Ploug, H.: High cell-specific rates of nitrogen and carbon fixation by the cyanobacterium Aphanizomenon sp. at low temperatures in the Baltic Sea, FEMS Microbiol. Ecol., 91, 1–11, https://doi.org/10.1093/femsec/fiv131, 2015.
Tan, J. W., Thong, K. L., Arumugam, N. D., Cheah, W. L., Lai, Y. W., Chua, K. H., Rahim, R. A., and Vikineswary, S.: Development of a PCR assay for the detection of nifH and nifD genes in indigenous photosynthetic bacteria, Int. J. Hydrogen Energ., 34, 7538–7541, https://doi.org/10.1016/j.ijhydene.2009.04.029, 2009.
Tang, W. and Cassar, N.: Data-Driven Modeling of the Distribution of Diazotrophs in the Global Ocean, Geophys. Res. Lett., 46, 12258–12269, https://doi.org/10.1029/2019GL084376, 2019.
Tang, W., Wang, S., Fonseca-Batista, D., Dehairs, F., Gifford, S., Gonzalez, A. G., Gallinari, M., Planquette, H., Sarthou, G., and Cassar, N.: Revisiting the distribution of oceanic N2 fixation and estimating diazotrophic contribution to marine production, Nat. Commun., 10, 831, https://doi.org/10.1038/s41467-019-08640-0, 2019.
Turk-Kubo, K. A., Karamchandani, M., Capone, D. G., and Zehr, J. P.: The paradox of marine heterotrophic nitrogen fixation: Abundances of heterotrophic diazotrophs do not account for nitrogen fixation rates in the Eastern Tropical South Pacific, Environ. Microbiol., 16, 3095–3114, https://doi.org/10.1111/1462-2920.12346, 2014.
Vahtera, E., Conley, D. J., Gustafsson, B. G., Kuosa, H., Pitkänen, H., Savchuk, O. P., Tamminen, T., Viitasalo, M., Voss, M., Wasmund, N., and Wulff, F.: Internal ecosystem feedbacks enhance nitrogen-fixing cyanobacteria blooms and complicate management in the Baltic Sea, Ambio, 36, 186–194, https://doi.org/10.1579/0044-7447(2007)36[186:IEFENC]2.0.CO;2, 2007.
Wacklin, P., Hoffmann, L., and Komárek, J.: Nomenclatural validation of the genetically revised cyanobacterial genus Dolichospermum (Ralfs ex Bornet et Flahault) comb, nova, Fottea, 9, 59–64, https://doi.org/10.5507/fot.2009.005, 2009.
Wannicke, N., Frey, C., Law, C. S., and Voss, M.: The response of the marine nitrogen cycle to ocean acidification, Glob. Change Biol., 24, 5031–5043, https://doi.org/10.1111/gcb.14424, 2018.
Wasmund, N.: Occurrence of cyanobacterial blooms in the baltic sea in relation to environmental conditions, Int. Rev. Gesamten Hydrobiol., 82, 169–174, https://doi.org/10.1002/iroh.19970820205, 1997.
Wasmund, N., Tuimala, J., Suikkanen, S., Vandepitte, L., and Kraberg, A.: Long-term trends in phytoplankton composition in the western and central Baltic Sea, J. Mar. Syst., 87, 145–159, https://doi.org/10.1016/j.jmarsys.2011.03.010, 2011.
Wulff, A., Karlberg, M., Olofsson, M., Torstensson, A., Riemann, L., Steinhoff, F. S., Mohlin, M., Ekstrand, N., and Chierici, M.: Ocean acidification and desalination: climate-driven change in a Baltic Sea summer microplanktonic community, Mar. Biol., 165, 1–15, https://doi.org/10.1007/s00227-018-3321-3, 2018.
Zani, S., Mellon, M. T., Collier, J. L., and Zehr, J. P.: Expression of nifH Genes in Natural Microbial Assemblages in Lake George, New York, Detected by Reverse Transcriptase PCR, Appl. Environ. Microbiol., 66, 3119, https://doi.org/10.1128/aem.66.7.3119-3124.2000, 2000.
Zehr, J. P., Mellon, M., Braun, S., Litaker, W., Steppe, T., and Paerl, H. W.: Diversity of heterotrophic nitrogen fixation genes in a marine cyanobacterial mat, Appl. Environ. Microbiol., 61, 2527–2532, https://doi.org/10.1128/aem.61.7.2527-2532.1995, 1995.
Zehr, J. P., Mellon, M. T., and Zani, S.: New nitrogen-fixing microorganisms detected in oligotrophic oceans by amplification of Nitrogenase (nifH) genes., Appl. Environ. Microbiol., 64, 3444–3450, 1998.
Zehr, J. P., Jenkins, B. D., Short, S. M., and Steward, G. F.: Nitrogenase gene diversity and microbial community structure: A cross-system comparison, Environ. Microbiol., 5, 539–554, https://doi.org/10.1046/j.1462-2920.2003.00451.x, 2003.
Zehr, J. P., Shilova, I. N., Farnelid, H. M., Muñoz-Maríncarmen, M. D. C., and Turk-Kubo, K. A.: Unusual marine unicellular symbiosis with the nitrogen-fixing cyanobacterium UCYN-A, Nat. Microbiol., 2, 16214, https://doi.org/10.1038/nmicrobiol.2016.214, 2016.
Zhang, X., McRose, D. L., Darnajoux, R., Bellenger, J. P., Morel, F. M. M., and Kraepiel, A. M. L.: Alternative nitrogenase activity in the environment and nitrogen cycle implications, Biogeochemistry, 127, 189–198, https://doi.org/10.1007/s10533-016-0188-6, 2016.