the Creative Commons Attribution 4.0 License.
the Creative Commons Attribution 4.0 License.
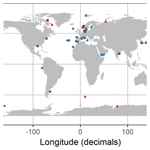
CO2 effects on diatoms: a synthesis of more than a decade of ocean acidification experiments with natural communities
Lennart Thomas Bach
Jan Taucher
Diatoms account for up to 50 % of marine primary production and are considered to be key players in the biological carbon pump. Ocean acidification (OA) is expected to affect diatoms primarily by changing the availability of CO2 as a substrate for photosynthesis or through altered ecological interactions within the marine food web. Yet, there is little consensus how entire diatom communities will respond to increasing CO2. To address this question, we synthesized the literature from over a decade of OA-experiments with natural diatom communities to uncover the following: (1) if and how bulk diatom communities respond to elevated CO2 with respect to abundance or biomass and (2) if shifts within the diatom communities could be expected and how they are expressed with respect to taxonomic affiliation and size structure. We found that bulk diatom communities responded to high CO2 in ∼60 % of the experiments and in this case more often positively (56 %) than negatively (32 %) (12 % did not report the direction of change). Shifts among different diatom species were observed in 65 % of the experiments. Our synthesis supports the hypothesis that high CO2 particularly favours larger species as 12 out of 13 experiments which investigated cell size found a shift towards larger species. Unravelling winners and losers with respect to taxonomic affiliation was difficult due to a limited database. The OA-induced changes in diatom competitiveness and assemblage structure may alter key ecosystem services due to the pivotal role diatoms play in trophic transfer and biogeochemical cycles.
- Article
(747 KB) - Full-text XML
- BibTeX
- EndNote
The global net primary production (NPP) of all terrestrial and marine autotrophs amounts to approximately 105 petagrams (Pg) of carbon per year (Field et al., 1998). Marine diatoms, a taxonomically diverse group of cosmopolitan phytoplankton, were estimated to contribute up to 25 % (26 Pg C yr−1) to this number, which is more than the annual primary production in any biome on land (Field et al., 1998; Nelson et al., 1995; Tréguer and De La Rocha, 2013). Thus, diatoms are likely the most important single taxonomic group of primary producers on Earth and any change in their prevalence relative to other phytoplankton taxa could profoundly alter marine food web structures and thereby affect ecosystem services such as fisheries or the sequestration of CO2 in the deep ocean (Armbrust, 2009; Tréguer et al., 2018).
The most conspicuous feature of diatoms is the formation of a silica shell, which is believed to primarily serve as protection against grazers (Hamm and Smetacek, 2007; Pančić and Kiørboe, 2018). Since the formation of this shell requires dissolved silicate, diatoms are often limited by silicon as a nutrient rather than by nitrogen or phosphate (Brzezinski and Nelson, 1996). However, when dissolved silicate is available, diatoms benefit from their high nutrient uptake and growth rates, allowing them to outcompete other phytoplankton and form intense blooms in many ocean regions (Sarthou et al., 2005).
Diatoms display an enormous species richness with recent estimates accounting for so far undiscovered diatoms (including freshwater) being in the range of 20 000–100 000 species (Guiry, 2012; Mann and Vanormelingen, 2013). Sournia et al. (1991) derive a number between 1400 and 1800 of described marine diatoms based on microscopy while Tara Oceans reported ∼4700 operational taxonomic units from genetic samples distributed over all major oceans except the North Atlantic and North Pacific (Malviya et al., 2016). Known diatom taxa span a size range of several orders of magnitude (< 5 µm up to a few millimetres) with a wide range of morphologies and life strategies, e.g. single cells, cell chains, and pelagic and benthic habitats (Armbrust, 2009; Mann and Vanormelingen, 2013; Sournia et al., 1991). Accordingly, they should not be treated as one functional group but rather as a variety of subgroups occupying different niches.
It is well recognized that the global importance of diatoms as well as their diversity in morphology and life style is tightly linked to the functioning of pelagic food webs and elemental cycling in the oceans. For example, iron enrichment experiments in the Southern Ocean found that a shift in diatom community composition from thick- to thin-shelled species (“persistence strategy” vs. “boom-and-bust strategy”) can enhance carbon and alter nutrient export via sinking particles (Assmy et al., 2013; Smetacek et al., 2012). This may not only affect element fluxes locally but also enhance nutrient retention within the Southern Ocean and reduce productivity in the north, which underlines how important diatom community shifts can be on a global scale (Boyd, 2013; Primeau et al., 2013; Sarmiento et al., 2004). Likewise, the cell size of diatoms can play an important role in transferring energy to higher trophic levels as the dominance of larger species is generally considered to reduce the length of the food chain and lead to higher trophic transfer efficiency (Sommer et al., 2002). Consequently, understanding impacts of global change on diatom community composition is crucial for assessing the sensitivity of biogeochemical cycles and ecosystem services in the world oceans.
It has become evident that the sensitivity of diatoms to increasing pCO2 is highly variable, likely being related to specific traits such as cell size or the carbon fixation pathway, as well as interactions with other environmental factors such as nutrient stress, temperature, or light (Gao et al., 2012; Hoppe et al., 2013; Wu et al., 2014). However, it is still rather unclear how these species-specific differences in CO2 sensitivities manifest themselves on the level of diatom communities. This knowledge gap motivated us to compile the presently available experimental data in order to reveal common responses of diatom communities to high CO2 and thereby assess potential scenarios of shifts in diatom community composition under ocean acidification.
2.1 Approach
Our original intention was to conduct a classical meta-analysis, which would have yielded the benefit of a quantitative measure of diatom responses to ocean acidification (OA), expressed as an overall effect size (i.e. combined magnitude) such as the response ratio. However, our literature analysis revealed a large variability in experimental pCO2 ranges as well as measured response variables, which cannot be directly compared among each other (e.g. microscopic cell counts, pigment concentrations, genetic tools). These limitations impede data aggregation as required for a classical meta-analysis. Furthermore, experimental setups differed widely in terms of other environmental factors such as temperature, light, and nutrient concentrations, all of which are known to modulate potential responses to pCO2 (Boyd et al., 2018), thereby further complicating data aggregation for meta-analysis. Therefore, we chose an alternative semi-quantitative approach where diatom responses to increasing CO2 are grouped in categories (see Sect. 2.2) and also allows us to account for differences in experimental setups, e.g. with respect to container volume (see Sect. 2.3). While this approach excludes the determination of effect size, it provides an unbiased insight into the direction of change of potential CO2 effects.
Before going into the details of data compilation we want to emphasize once more that the motivation for this study was not to investigate the physiological response of diatoms to OA. Such meta-analyses or reviews have already been made (Dutkiewicz et al., 2015; Gao and Campbell, 2014). Instead, our goal was to summarize how diatoms respond to OA in their natural habitat. More generally, experiments with ecological communities (as compiled in our study) do not so much aim for a mechanistic understanding of a certain process (e.g. in physiological experiments) but rather assess the general sensitivity of more natural communities to environmental drivers. Therefore, it is important to have a realistic setup because the net response of any player in the food web is composed of a direct physiological response to CO2 and by CO2-induced alterations of interactions with other species. From that point of view it is desirable to include all important ecosystem components because when trophic cascades are represented incompletely then the observed response in an experiment may not reflect the response that would occur in nature, which is what we are ultimately interested in (Carpenter, 1996). Clearly, investigating OA effects on diatoms or any other group in complex communities has the disadvantage that the actual cause for an observed response can hardly ever be determined with high certainty (Bach et al., 2017, 2019). However, experiments compiled herein investigated the development of initially similar plankton communities over time with the only difference being carbonate chemistry conditions between control and the treatments. Thus, we can at least be sure that the differences in diatom abundance or community composition between control and treatment (which is the focus of our study) is caused by simulated OA, even though the underlying mechanisms cannot be pinned down with certainty.
2.2 Data compilation
We explored the response of diatom assemblages to high CO2 (low pH) by searching the literature for relevant results with Google Scholar (15 December 2017) using the following search query: “diatom” OR “Bacillariophyceae” AND “ocean acidification” OR “high CO2” or “carbon dioxide” OR “elevated CO2” OR “elevated carbon dioxide” OR “low pH” OR “decreased pH”. The first 200 results were inspected and considered to be relevant when they were published in peer-reviewed journals contained a description of the relevant methodological details, a statistical analysis or at least a transparent description of variance and uncertainties, and tested CO2 effects on natural plankton assemblages (artificially composed communities were not considered). We then carefully checked the cited literature in these relevant studies to uncover other studies that were missed by the initial search. Furthermore, we checked the “Ocean acidification news stream” provided by the “Ocean Acidification International Coordination Centre” under the tag “phytoplankton” (https://news-oceanacidification-icc.org/tag/phytoplankton/, last access: 16 January 2019) for relevant updates since December 2017.
There were two response variables of interest for the literature compilation:
-
The response of the “bulk diatom community” to high CO2. For this we checked if the abundance of diatoms, the biomass of diatoms, or the relative portion of diatoms within the overall phytoplankton assemblage increased or decreased under high CO2 relative to the control. We distinguished between “positive”, “negative”, and “no effect” following the statistical results provided in the individual references. When the CO2 effect on the bulk community was derived from abundance data, we also checked if there were indications for a concomitant shift in the biomass distribution among species. This is relevant because, for example, an increase in bulk abundance could coincide with a decrease in bulk biomass when the species driving the abundances is smaller. We found no indications for conflicting cases but acknowledge that not every reference provided sufficient data on morphological details to fully exclude this scenario. Furthermore, we emphasize that CO2 can also shift the temporal occurrence of a diatom response (Bach et al., 2017). For example, a diatom bloom could occur earlier in a high CO2 treatment than in the control but with a similar bloom amplitude (Donahue et al., 2019). In this case we assigned a “positive” response because an earlier bloom occurrence mirrors a higher net growth rate under elevated CO2.
-
The CO2-dependent species shifts within the diatom community with respect to taxonomic composition and/or size structure. Unfortunately, cell size of the species was not reported for all experiments. Thus, we distinguished between “no shifts”, “shifts between species with unspecified size”, and “shifts towards larger or smaller species” when this information was provided. Furthermore, we noted the winners and losers within the diatom communities when these were reported (on the genus level).
In the case when the data were taken from factorial multiple stressor experiments (e.g. CO2 × temperature), we considered only the control conditions with respect to the stressors other than CO2 (e.g. at control temperature). Furthermore, we extracted various metadata from each study largely following the literature analysis of Schulz et al. (2017). All bulk diatom responses, community shifts, and metadata are compiled and described in Table 1 and most of it is self-explanatory (e.g. incubation temperature). The coordinates from where the investigated plankton communities originate are given in Table 1 and illustrated in Fig. 2. Their habitats were categorized according to water depth, salinity, or life style in the case of benthic communities: “oceanic” means water depth > 200 m (unless the habitat lies within a fjord or fjord-like strait), S > 30; “coastal” means water depth < 200 m, S > 30; “estuarine” means water depth < 200 m, S < 30; and “benthic” means benthic communities (diatoms growing on plates) were investigated. We reconstructed the water depth in case it was not provided in the paper using Google Earth Pro (version 7.3.2.5495). The coordinates provided in some of the experiments conducted in land-based facilities were imprecise and marked positions on land. In this case the habitats were set to coastal or estuarine depending on salinity. If salinity was not given we checked the location on Google Earth for potential fresh water sources and also checked the text for more cryptic indications (e.g. “euryhaline” in a lagoon were strong indications for an estuarine habitat). The methods with which responses of the bulk diatom communities to high OA were determined varied greatly among studies and included light microscopy (LM), pigment analyses (PA), flow cytometry (FC), genetic tools (e.g. PCR), and biogenic silica (BSi) analyses (Table 1).
Table 1Response of diatom communities to high CO2. A total of 69 experiments from 54 publications were considered. Lat and long refer to the coordinates where diatom communities were collected. The RDR (relative degree of realism) is dimensionless (see Sect. 2.3). S is the average salinity of incubations. T is the average incubation temperature in degrees Celsius (∘C). Habitats were determined as described in section 2.2 (est. is estuarine). DoE are days of experiment with the number (no.) of samplings given as the second number. Pre-filt. gives the mesh size when the collected plankton community was pre-filtered before incubation. No means no pre-filtration treatment. Setup refers to the incubation style: undiluted volumes (batch), repeatedly diluted volumes (s.-cont.), flow-through setups (fl.-thr.; only benthos), chemostats (chem.; only pelagic), and CO2 vent sites (seep; only benthos). Incubations (Incub.) can either be performed on deck (e.g. shipboards), in situ (e.g. in situ mesocosms), or under laboratory conditions. V refers to the incubation volume. Nutrient amendments (Nutr.) were made in some but not all studies. The element indicates which nutrients were added. Asterisks indicate the presence of residual nutrients at the beginning of the study. Manipulations (Manip.) were done with CO2-saturated seawater (SWsat), acid additions (Acid), combined additions of acid and base (Comb.), CO2 gas additions (CO2), aeration at target pCO2 (Aer.), and passing CO2 gas through a diffusive silicone tubing (Diff.). Meth. indicates the applied methodology to investigate diatom communities: light microscopy (LM), pigment analyses (PA), flow cytometry (FC), genetic tools (e.g. PCR), and biogenic silica (BSi). The pCO2 range of the experiment with the number of treatments is given in brackets. The response of the bulk diatom community to CO2: no effect (∼ ), positive (p), negative (n), or not reported (N/A). The pCO2 response indicates approximately in between which treatments a CO2 response was observed. Please note that this is based on visual inspection of the datasets and therefore involves subjectivity. Please also note that the range equals the treatment values when only two treatments were set up. CO2-induced shifts between diatom species can shift to larger species (large), shift to smaller species (small), unspecified shift (shift), no species shift detected (∼), or not reported (N/A). Winners or losers of the diatom community comprise Chaetoceros (Chae), large Chaetoceros (Chae I), medium Chaetoceros (Chae II), small Chaetoceros (Chae III), Neosyndra (Neos), Rhabdonema (Rhab), Eucampia (Euca), Cerataulina (Cera), Thalassiosira (Thals), Proboscia (Prob), Pseudo-nitzschia (Ps-n), Thalassionema (Thaln), Cylindrotheca (Cyli), Guinardia (Guin), Synedropsis (Syned), Dactyliosolen (Dact), Toxarium (Toxa), Leptocylindrus (Lept), Grammatophora (Gram), Bacillaria (Baci), or Navicula (Navi).
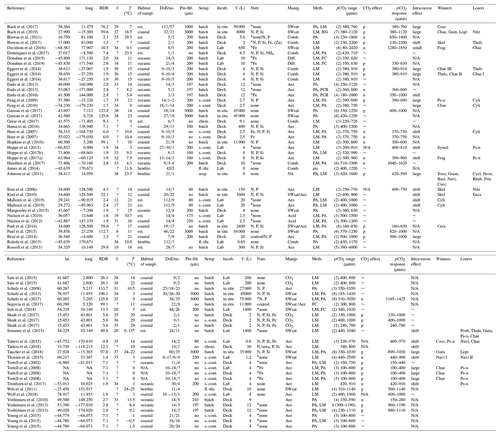
* They used 9 and 15 ∘C as incubation temperature but neither were control treatments.
2.3 Accounting for different experimental setups to balance the influence of individual studies on the outcome of the literature analysis
The most realistic OA experiment would be one where all aspects of the natural habitat are represented correctly. Such setups are possible for benthic communities which can be sampled in situ along a natural CO2 gradient at volcanic CO2 seeps (Fabricius et al., 2011; Hall-Spencer et al., 2008; Johnson et al., 2011). However, pelagic communities are advected with currents so that it is very difficult to simulate OA in open waters. Thus, OA experiments where pelagic communities are exposed to increasing levels of CO2 were so far always performed in closed containers even though it is well known that confinement causes experimental artefacts (Calvo-Díaz et al., 2011; Ferguson et al., 1984; Guangao, 1990; Menzel and Case, 1977). The degree by which confinement causes experimental artefacts will differ from study to study, depending on factors such as the incubation volume, the length of incubation, or the selective removal of certain size classes from the incubation (Carpenter, 1996; Duarte et al., 1997; Nogueira et al., 2014). In our literature synthesis we had to deal with a large variety of experimental setups and there are very likely differences in how well a given setup represents the natural environment. Therefore, we aimed to develop a metric that allows us to estimate “how well the natural system (which we are ultimately interested in) is represented by the experimental setup”. This metric – termed the “relative degree of realism (RDR)” – was used to balance the influence of individual studies on the final outcomes of the literature analysis. Most certainly, we do not mean to devalue any studies but think that the highly different scales of experiments, ranging from 0.8 L lab incubations to 75 m3 in situ mesocosms, should not be ignored when evaluating the literature. In the following we will first derive the equation for the RDR and introduce the underlying assumptions. Afterwards we describe aspects that were considered while conceptualizing the RDR.
The incubation volume in the studies considered herein ranged from bottle experiments to in situ mesocosm studies with considerably larger incubation volumes. Smaller differences in incubation volumes (e.g. 0.5 vs. 2 L) were shown to have no, or a minor, influence on physiological rates (Fogg and Calvario-Martinez, 1989; Hammes et al., 2010; Nogueira et al., 2014; Robinson and Williams, 2005). However, they can influence food web composition (Calvo-Díaz et al., 2011; Spencer and Warren, 1996), e.g. by unrepresentatively including certain organism groups such as highly motile mesozooplankton. Larger differences in incubation volumes (e.g. 10 vs. 10 000 L) are considered to have a major influence on the enclosed communities, with the larger volume generally being more representative of natural processes (Carpenter, 1996; Duarte et al., 1997; Sarnelle, 1997). Therefore, our first assumption to conceptualize the RDR was that larger incubation volumes represent nature generally better than smaller ones.
Plankton communities were pre-filtered in many experiments to exclude larger and often patchily distributed organisms (e.g. copepods). This is a valid procedure to reduce noise and to increase the likelihood to detect CO2 effects but it also influences the development of plankton communities since the selective removal of certain size classes can modify trophic cascades within the food web (Ferguson et al., 1984; Nogueira et al., 2014). For example, Nogueira et al. (2014) compared plankton successions of pre-filtered (100 µm) and unfiltered communities and found that the removal of larger grazers and diatoms gave room for green algae and picophytoplankton to grow. Such manipulations make the experiment less representative for a natural food web, which brought us to the second assumption for the RDR: the smaller the mesh size during the pre-filtration treatment, the less complete and thus the less realistic is the pelagic food web.
To parameterize the two abovementioned assumptions, we first converted the volume information provided in each experiment into a volume-to-surface ratio (V∕S). The underlying thought is that V increases with the third power to the surface area of the incubator and is indicative of the relation of open space to hard surfaces. Therefore, we first converted V into a radius (r) assuming spherical shape:
The surface (S) of the spherical volume was calculated as
The assumption of spherical shape was necessary because it allowed us to calculate V∕S from only knowing V, which is usually the only parameter provided with respect to container characteristics. We are aware that this is a simplification because the majority of containers used in experiments will likely have had cylindrical shape. However, the conversion from volume to surface assuming cylindrical shape would have required knowledge of two dimensions (radius and height of the cylinder). Although shape can influence processes within the container (Pan et al., 2015), it is a less important factor to consider in our study because sensitivity calculations assuming reasonable cylinder dimensions showed that the V∕S differences due to container shape will be small compared to the V∕S differences due to the range of container volumes compared here.
The influence of pre-filtration treatments on the investigated plankton community is implemented by multiplying the V∕S with the cube root of the applied mesh size (dmesh in microns,µm) so that the RDR is defined as
Thus, as for V∕S, the influence of dmesh on RDR does not increase linearly but becomes less influential with increasing dmesh. The rationale for the non-linear increase is that incubations will still have an increasing bias even if they do not have any pre-filtration treatment due to generally increasing organism motility with size. For example, when collecting a plankton community with a Niskin bottle, more motile organisms can escape from the approaching sampler so that the food web composure is still affected even without subsequent pre-filtration. For this reason, we also capped the maximum dmesh to 10 000 µm when there was no pre-filtration treatment applied since none of the studies included significantly larger organisms. The rationale for calculating the cube root of dmesh was that in this case the influence of V∕S and dmesh on RDR becomes roughly similar. Figure 1 illustrates the change of RDR as a function of V and dmesh. High RDRs are calculated for large-scale in situ mesocosm studies (∼50–190), while bottle experiments yield RDRs between ∼1 and 12.
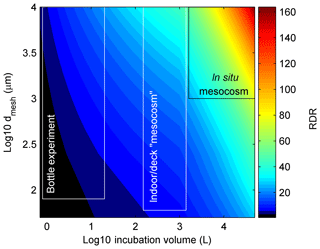
Figure 1RDR as a function of incubation volume and size of the mesh that was used while filling the incubation volumes (dmesh). The black and white boxes illustrate approximate ranges of the three main types of containers used in experiments. Please note that the general definition for mesocosms are volumes ≥1000 L (Guangao, 1990) but since most authors also use this term for open batch incubations with volumes between 150–1000 L we also stick to this term for the intermediate class.
The key pre-requisite for an experimental parameter to be included in the RDR equation (Eq. 3) was that it is reported in all studies. Many parameters that we would have liked to use for the RDR are either insufficiently reported (e.g. the light environment) or not provided quantitatively at all (e.g. turbulence). We therefore had to work with very basic properties related to the experimental setup rather than to the experimental conditions.
A particularly critical aspect of the RDR we had to deal with was the duration of the experiments (Time). Time is reliably reported in all studies and therefore principally suitable for the RDR. Our first thoughts were that a realistic community experiment should be long enough to cover relevant ecological processes such as competitive exclusion and therefore also parameterized Time in the first versions of the RDR equation. However, we decided to not account for it in the final version because the factors that define the optimal duration of an experiment are poorly constrained. For example, a 1 d experiment in a 10 L container could indeed miss important CO2 effects caused by food web interactions. On the other hand, a 30 d experiment in the same container could reveal such indirect effects but at the same time be associated with profound bottle effects and make the study unrepresentative for simulated natural habitat. Thus, too long and too short times are both problematic and the optimum is hard to find. One such attempt to find the optimum Time was made by Duarte et al. (1997), who analysed the plankton ecology literature between 1990 and 1995. By correlating the experimental duration with the incubation volume of published experiments they provided an optimal length for any given volume. However, as noted by Duarte et al. (1997), their correlation is based on publication success and therefore rather reflects common practice in plankton ecology experiments and not necessarily a mechanistic understanding of bottle effects. Thus, as there is no solid ground for a parameterization of Time we ultimately decided to not consider it for the RDR.
Finally, we want to point out (and explicitly acknowledge) that the RDR approach to balance the influence of studies on the final outcome of the literature analysis is of course not the one perfect solution and most likely incomplete (see above). However, balancing a literature analysis with the RDR score may still be an improvement relative to the other case where each experiment is treated exactly equally despite huge differences in the experimental setup. Nevertheless, to account for both views (i.e. the RDR is useless vs. the RDR is useful) we will present the outcome of our literature analysis in two different ways throughout the paper: (1) by simply counting the number of outcomes (N) and adding them to yield a cumulative ∑N score (N-based approach; left columns in Figs. 3 and 4) or (2) by adding the RDR score of the experiments with a certain outcome to yield a cumulative ∑RDR score (RDR-based approach; right columns in Figs. 3 and 4).
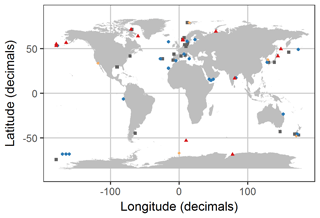
Figure 2Distribution of experiments with associated OA response of the bulk diatom communities as listed in Table 1. Blue circles indicate positive response; red triangles indicate a negative response; grey squares indicate no response; orange diamonds indicate a response with unknown direction of change. Locations were slightly modified in case of geospatial overlap to ensure visibility. Please note that the three blue points in the Ross Sea at about −68, −165 are approximate locations because the reference did not provide coordinates.
We found 54 relevant publications on CO2 experiments with natural diatom assemblages. Some publications included more than one experiment so that 69 experiments are considered hereafter (Table 1). Most were done with plankton communities from coastal (46 %) and oceanic (28 %) environments. Estuarine and benthic communities were investigated in 16 % and 6 % of the studies, respectively. And 4 % of the studies did not provide coordinates where the samples were taken although the region was reported (Table 1; Fig. 2).
Among the 69 experiments, 23 (33 %, ∑RDR =595) revealed a positive influence of CO2 on the “bulk diatom community” (see Sect. 2.2), while 13 (19 %, ∑RDR =266) revealed a negative one; 5 experiments (7 %, ∑RDR =21) found a CO2 effect but did not specify whether it is a positive or negative one; and 28 experiments (41 %, ∑RDR =728) found no effect (Fig. 3a).
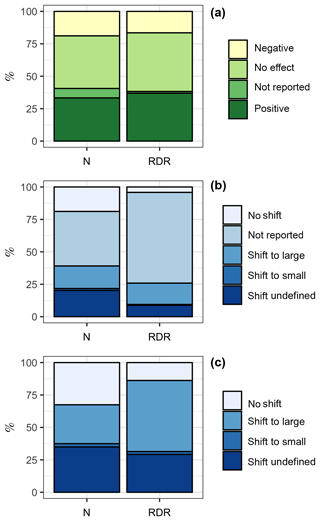
Figure 3Summary of the literature analysis. (a) Response of the bulk diatom community to ocean acidification. (b) Shifts among different diatom species due to ocean acidification. “Shift to large” and “shift to small” indicate that the diatom community shifted towards the dominance of larger or smaller species, respectively. (c) Same data as in (b) but excluding studies where species shifts within the diatom community were not reported. This reduced the dataset from 69 to 40 studies. The left column is based on the number of studies. For example, the bulk diatom community was positively affected by OA in 23 out of 69 studies, which is 33 %. The right column is based on the RDR values. For example, the ∑RDR value of all studies where the diatom community was positively affected by OA was 595, which is 37 % of the total ∑RDR. Please keep in mind that the RDR-based approach excludes benthic studies, whereas the N-based approach includes them.
We also checked if the pCO2 range tested in the experiments had an influence on whether the bulk diatom community responded to changing carbonate chemistry. This was done because we expected the likelihood to find an OA response to be higher when the pCO2 difference between treatments and controls is larger. Thus, we calculated the investigated pCO2 range (highest pCO2 – lowest pCO2) for each experiment and categorized the range into “small” (≤300 µatm), “medium (300–600 µatm), and “large” (≥600 µatm). Among the 41 experiments that found a CO2 effect on the bulk diatom community (positive, negative, and unreported direction of change), 4 (10 %, ∑RDR =106) found it within the low range, 12 (29 %, ∑RDR =123) in the medium range, and 25 experiments (61 %, ∑RDR =653) in the high range. Among the 28 experiments that found no CO2 on the bulk diatom community, 3 (11 %, ∑RDR =12) tested within the low range, 8 (29 %, ∑RDR =230) within the medium range, and 17 experiments (61 %, ∑RDR =487) within the high range. According to this analysis, the likelihood of detecting a CO2 effect on the bulk diatom community does not depend on the investigated pCO2 range.
CO2-dependent shifts in diatom species composition were investigated with light microscopy except for Endo et al. (2015), who used molecular tools. Species shifts were investigated in a subset of 40 of the 69 experiments (Fig. 3b). Within this subset of 40 studies, 12 (30 %, ∑RDR =265) found a shift towards larger diatom species under high CO2, 1 (2.5 %, ∑RDR =10) found a shift towards smaller diatom species, and 13 (32.5 %, ∑RDR =67) found no CO2 effect on diatom community composition. Fourteen studies (35 %, ∑RDR =141) reported a CO2-dependent shift but did not further specify any changes in the size-class distribution (Fig. 3c).
We also tested if the bulk diatom response to OA in coastal, estuarine, and benthic environments was different from the bulk response in oceanic environments. The rationale for this comparison was that carbonate chemistry conditions in oceanic environments may generally be more stable than in the often more productive coastal, estuarine, and benthic environments (Duarte et al., 2013; Hofmann et al., 2011). Therefore, diatoms from oceanic environments may be more sensitive to OA (Duarte et al., 2013). We found 47 experiments with coastal + estuarine + benthic diatom communities. Within this subset, 15 experiments (32 %, ∑RDR =557) revealed a positive influence of CO2 on the “bulk diatom community” while 6 (13 %, ∑RDR =244) revealed a negative one. Four experiments (9 %, ∑RDR = 19) found a CO2 effect but did not specify whether it is a positive or negative one. Twenty-two experiments (47 %, ∑RDR =715) found no effect (Fig. 4a). In contrast, we found 19 experiments with oceanic communities. Within this subset, 5 experiments (26 %, ∑RDR =17) revealed a positive influence of CO2 on the “bulk diatom community” while 7 (37 %, ∑RDR =21) revealed a negative one. One experiment (5 %, ∑RDR =2) found a CO2 effect but did not specify whether it is a positive or negative one. Six experiments (32 %, ∑RDR =13) found no effect (Fig. 4b). Overall, we found a bulk diatom response to OA (positive, negative, and unreported direction of change) in 53 % of the experiments in coastal + estuarine + benthic environments as opposed to 68 % in oceanic environments. Thus, an OA response to the bulk diatom community was more frequently observed in oceanic environments, which was mostly due to the higher frequency of negative OA responses (Fig. 4).
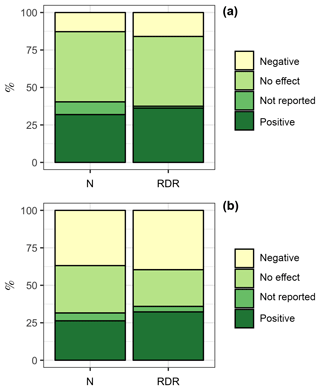
Figure 4Comparison of the diatom bulk response to OA in different environments. (a) Coastal + estuarine + benthic environments with 47 experiments. (b) Oceanic environments with 19 experiments. The left column is based on the number of studies. For example, the bulk diatom community was positively affected by OA in 5 out of 19 studies in oceanic environments, which is 26 %. The right column is based on the RDR values. For example, the ∑RDR value of all studies where the oceanic diatom community was positively affected by OA was 17, which is 32 % of the total ∑RDR. Please keep in mind that the RDR-based approach excludes benthic studies, whereas the N-based approach includes them.
Numerous physiological studies have shown that diatom growth and metabolic rates can be affected by seawater CO2 concentrations and that these responses vary widely among different species (Gao and Campbell, 2014). Such inter-specific differences in pCO2 sensitivity are an important feature as this could alter the composition of diatom assemblages in a changing ocean. In this regard, it is interesting to note that paleolimnologists have long been using diatom species composition as palaeo-proxy to reconstruct lake pH (Battarbee et al., 2010). Hence, there is ample evidence that high CO2 conditions have the potential to change the diatom species composition.
Indeed, our analysis revealed that CO2-induced changes in diatom community composition occurred in 27 out of 40 (i.e. 68 %) of community-level experiments which investigated species composition (Fig. 3c). This is certainly a conservative outcome because many studies have only looked at dominant species. In fact, one of the few experiments that investigated the diatom assemblage with higher taxonomical resolution found CO2 effects also on sub-dominant species (Sommer et al., 2015), which may have been overlooked in many other experiments.
The comparison of OA effects in different environments revealed that bulk diatom communities responded more frequently to OA in oceanic than in coastal + estuarine + benthic environments. Especially negative effects of OA were more frequent in oceanic environments (Fig. 4). This result is not particularly surprising since communities found near coasts may be adapted to larger carbonate chemistry variability (Duarte et al., 2013) and therefore be better suited to deal with OA. It should be kept in mind, however, that this comparison is based on only 19 oceanic experiments in contrast to 47 coastal + estuarine + benthic experiments. Furthermore, our habitat characterization depends on certain criteria (mainly water depth and salinity; see Sect. 2.2) and these may be insufficient for our habitat comparison. For example, plankton communities from near oceanic islands such as the Azores were labelled as “coastal” although they may have been moving within oceanic currents and just happened to be close to shore when they were collected. Accordingly, this type of habitat comparison would be more robust if the community had been characterized based on the prevailing carbonate chemistry they are usually exposed to. Unfortunately, information on the background carbonate chemistry is hardly ever provided.
4.1 CO2 effects on diatom assemblages originating from (direct) physiological responses to high CO2
Most studies that found effects of pCO2 on diatom communities related these changes to CO2 fertilization of photosynthesis. Concentrations of CO2 in the surface ocean are relatively low compared to other forms of inorganic carbon, especially bicarbonate ion () (Zeebe and Wolf-Gladrow, 2001). However, RuBisCO, the primary carboxylating enzyme used in photosynthesis, is restricted to CO2 for carbon fixation and has a relatively low affinity for CO2 compared to O2 (Falkowski and Raven, 2007). Therefore, diatoms (like many other phytoplankton species) operate a carbon concentrating mechanism (CCM) to enhance their CO2 concentration at the site of fixation relative to external concentrations (e.g. by converting to CO2) and thereby establish higher rates of carbon fixation than what would be possible when only depending on diffusive CO2 uptake (Giordano et al., 2005). It is well known that the proportion of CO2 uptake vs. uptake for photosynthesis varies largely among diatoms (Burkhardt et al., 2001; Rost et al., 2003; Trimborn et al., 2008) and is theoretically also a function of cell size (Flynn et al., 2012; Wolf-Gladrow and Riebesell, 1997). Accordingly, increasing seawater pCO2 may increase the proportion of diffusive carbon uptake and/or lower the energy and resource requirements for CCM operation (Raven et al., 2011). From a physiological point of view, these mechanisms could allow for increased rates of photosynthesis and cell division.
So how do these theoretical considerations align with (a) the variable and species-specific physiological responses of diatoms to increasing CO2 (Dutkiewicz et al., 2015) and (b) the results from community-level experiments compiled in this study? Regarding the variability of physiological responses, progress has recently been made by Wu et al. (2014), who experimentally demonstrated a positive relationship between cell volume and the magnitude of the CO2 fertilization effect on diatom growth rates. Their findings agree well with theoretical considerations, which predict that high CO2 is particularly beneficial for carbon acquisition by larger species as they are more restricted by diffusion gradients due to lower surface-to-volume ratios than smaller cells (Flynn et al., 2012; Wolf-Gladrow and Riebesell, 1997). The outcome of our literature analysis supports this allometric concept (Fig. 3, Table 2). Twelve out of 13 experiments in which cell size was taken into account found a shift towards larger species. This is reflected in the ∑RDR score of 265 which is ∼25 times higher than the opposite result (i.e. CO2-induced shifts towards smaller diatoms, Fig. 3c). An allometric scaling of CO2 sensitivity is particularly useful for modelling since cell size is a universal trait which is relatively easy to measure and therefore frequently available (Ward et al., 2012). Accordingly, it may lead to significant improvements of ecological and/or biogeochemical model projections under CO2 forcing when more than one size class for diatoms is considered.
However, although the Wu et al. (2014) allometric approach constitutes a solid starting point to help with understanding the variable responses of different diatom species, it probably also still needs some further refinements. For example, central components of CCMs seem to be adapted to diatom cell sizes, thereby potentially alleviating a strict cell size dependency of CO2 limitation (Shen and Hopkinson, 2015). Furthermore, size dependency alone cannot account for taxon-specific differences in the mode of carbon acquisition (diffusive uptake of CO2 vs. CCM-supported uptake of ) and how this will affect the competitive ability of species under increasing CO2. OA will lead to much larger changes in dissolved CO2 than in . Thus, species that rely to a larger extent on a resource-intensive CCM may benefit more from increasing pCO2 on a cellular level as they could increase the proportion of diffusive CO2 uptake. However, it is also possible that the same species would be disadvantaged on the community-level because their niche, i.e. being competitive at lower CO2 due to an efficient CCM, is diminished under high CO2 conditions (a scenario that is neglected in the physiological literature). Which of the scenarios occurs in nature would also depend on how flexible species are in terms of switching carbon acquisition modes, as well as resource allocation. In this regard, it is noteworthy that only few physiological studies on OA effects have taken into account the role of changing nutrient concentrations or even a transition to nutrient limitation. The available experimental evidence suggests that increasing pCO2 may reduce cellular nutrient requirements for CCM operations and therefore free resources for elevated maximum diatom population densities, particularly when running into nutrient limitation (Taucher et al., 2015). Unfortunately, however, the relevance of this mechanism has so far only been investigated in mono-clonal laboratory experiments but not on the community level.
These considerations illustrate that cell size is an important factor but is not sufficient to predict physiological or even the community level of diatoms to OA. Moreover, the allometric concept as well as the additional mechanisms described above generally presumes positive effects of CO2 fertilization, thus yielding no first-order explanations for observed negative responses of diatoms to changing carbonate chemistry. Obviously, increasing CO2 concentrations are accompanied by increasing proton (H+) concentrations under ocean acidification. High H+ concentrations may reduce key metabolic rates above certain thresholds and outweigh the positive influence of CO2 fertilization as has been observed in coccolithophores (Bach et al., 2011, 2015; Kottmeier et al., 2016).
Another pathway by which ocean acidification may alter diatom communities is the pH effect on silicification and silica dissolution. Low seawater pH should theoretically facilitate silicification as the precipitation of opal occurs in a cellular compartment with low-pH conditions (pH ∼5) (Martin-Jézéquel et al., 2000; Vrieling et al., 1999). At the same time, a lower pH should reduce chemical dissolution rates of the SiO2 frustule (Loucaides et al., 2012). While experimental evidence on this topic is still scarce and partly controversial (Hervé et al., 2012; Mejía et al., 2013; Milligan et al., 2004), it is not unlikely that OA-induced changes in the formation and dissolution of biogenic silica may alter the strength of the frustule and therefore the palatability of diatoms to zooplankton grazers (Friedrichs et al., 2013; Hamm et al., 2003; Liu et al., 2016; Wilken et al., 2011). As for the other physiological effects, e.g. carbon fixation, it is likely that OA impacts on silicification will vary among different diatom species, e.g. according to their species-specific intrinsic buffering capacity, thereby leading to further taxonomic shifts within diatom communities.
The response of diatoms to increasing pCO2 in natural environments will be further modified by multiple other environmental drivers changing simultaneously. Climate change is expected to elevate ocean temperature, as well as also irradiance and nutrient availability via changes in stratification. Physiological experiments have shown that elevated pCO2 may have beneficial effects under low and moderate irradiance but this effect may reverse under high light conditions due to enhanced photoinhibition (Gao et al., 2012). Analogously, warming may have positive or negative effects on photosynthesis and metabolism in general, depending on the thermal optima of the respective species (Boyd et al., 2018). Altogether, these multiple additional drivers will also affect diatom communities, leading to shifts in their taxonomic composition and size structure, which will interact with the impacts of OA.
4.2 Indirect CO2 effects on diatom assemblages through food web interactions
Diatom community responses cannot only originate from a direct CO2 effect on their physiology but also be caused indirectly through CO2 responses on other components of the food web (Bach et al., 2017; Gaylord et al., 2015). For example, if a grazer of a diatom species is negatively affected by OA then this may benefit the prey and indirectly promote its abundance. Direct OA impacts on zooplankton communities are usually assumed to play a minor role, although there is some experimental evidence that lower pH may have physiological effects at least on some sensitive species or developmental stages (Cripps et al., 2016; Thor and Dupont, 2015; Thor and Oliva, 2015). Nevertheless, much of the currently available empirical evidence indicates that zooplankton communities are affected by OA rather via bottom-up effects, e.g. via changes in primary production or taxonomic composition of the phytoplankton community (Alvarez-Fernandez et al., 2018; Meunier et al., 2017; Sswat et al., 2018). However, bottom-up effects on zooplankton biomass, size structure, or species composition may in turn trigger feedbacks on diatom communities, thereby leading to a feedback loop that may reinforce until a new steady state is reached. Such considerations illustrate that also second- or third-order effects need to be considered when assessing OA effects on the level of ecological communities. Accounting for such indirect effects requires a holistic approach considering all key players in of the food web (something that is beyond the scope of this study). Therefore, interpretations about what the observed responses could mean for entire plankton food webs or even biogeochemical element cycles (Sect. 4.3) should always be regarded with some healthy scepticism as they often neglect the potential for indirect effects.
4.3 Implications of changes in diatom community structure for pelagic food webs and biogeochemical cycles
The taxonomic composition and size structure of phytoplankton communities influences the transfer of energy from primary production to higher trophic levels. In theory, larger diatoms should support a more direct transfer because less trophic intermediates are needed and therefore less respiration occurs until prey items are in an appropriate size range for top predators (Azam et al., 1983; Pomeroy, 1974; Sommer et al., 2002). Such a size shift at the bottom of a food web might eventually lead to higher production in higher trophic levels such as fish. Indeed, recent experimental evidence indicated that fish (including commercially important species) could, under certain constellations, benefit from high CO2 due to higher food availability, although it was not tested if this response is somehow linked to the diatom size structure (Goldenberg et al., 2018; Sswat et al., 2018).
Fluxes of elements through the oceans are (like fluxes of energy through food webs) influenced by the composition of diatom communities (Tréguer et al., 2018). This is particularly well recognized in the context of organic carbon export to the deep ocean, for which diatoms are considered to play a pivotal role (Smetacek, 1985). Given that high CO2 favours large and perhaps more silicified diatoms over smaller ones (Sect. 4.1), we might expect accelerated sinking and thus a positive feedback on the vertical carbon flux. This classical hypothesis is supported by observational evidence from two consecutive years of the North Atlantic spring bloom where, despite similar primary production, particulate organic carbon sequestration into the deep ocean (3100 m) was much higher in the year when the larger diatom species dominated (Boyd and Newton, 1995). However, whether the positive relationship between size and carbon export holds under all circumstances is by no means clear (Tréguer et al., 2018). It is possible that shifts towards larger-sized species coincide with shifts in other traits that feed back negatively on carbon export. For example, when the size shift is associated with decreasing C : Si stoichiometry it may ultimately reduce carbon export (Assmy et al., 2013).
The abovementioned examples of trophic transfer and export fluxes illustrate the importance of the factor “diatom community structure” in the context of marine food production and biogeochemical fluxes. They also illustrate that our understanding of the feedbacks induced through changes in diatom communities is highly incomplete. Hence, with our limited understanding we currently cannot go further than classifying CO2-induced changes in diatom communities as “a potential risk” that may cause changes in key ecosystem services.
All data used in this study are compiled in Table 1.
LTB did the literature analysis, conceptualized the RDR, and drafted the article, except for parts of the introduction and discussion. JT drafted parts of the introduction and discussion. Both authors interpreted the findings and revised the article.
The authors declare that they have no conflict of interest.
We thank Nauzet Hernández-Hernández, Ulf Riebesell, and Javier Arístegui for their comments on an earlier version of the data compilation. The research was funded by the Federal Ministry of Science and Education (Bundesministerium für Bildung und Forschung; BMBF) in the framework of the “Biological Impacts of Ocean Acidification” project (BIOACID III, FKZ 03F0728) as well as GEOMAR Helmholtz Centre for Ocean Research Kiel and the Australian Research Council within a laureate (FL160100131) granted to Philip Boyd.
The article processing charges for this open-access publication were covered by a Research Centre of the Helmholtz Association.
This paper was edited by Piers Chapman and reviewed by two anonymous referees.
Alvarez-Fernandez, S., Bach, L. T., Taucher, J., Riebesell, U., Sommer, U., Aberle, N., Brussaard, C. P. D., and Boersma, M.: Plankton responses to ocean acidification: The role of nutrient limitation, Prog. Oceanogr., 165, 11–18, https://doi.org/10.1016/j.pocean.2018.04.006, 2018.
Armbrust, E. V.: The life of diatoms in the world's oceans, Nature, 459, 185–192, https://doi.org/10.1038/nature08057, 2009.
Assmy, P., Smetacek, V., Montresor, M., Klaas, C., Henjes, J., Strass, V. H., Arrieta, J. M., Bathmann, U., Berg, G. M., Breitbarth, E., Cisewski, B., Friedrichs, L., Fuchs, N., Herndl, G. J., Jansen, S., Kragefsky, S., Latasa, M., Peeken, I., Rottgers, R., Scharek, R., Schuller, S. E., Steigenberger, S., Webb, A., and Wolf-Gladrow, D.: Thick-shelled, grazer-protected diatoms decouple ocean carbon and silicon cycles in the iron-limited Antarctic Circumpolar Current, P. Natl. Acad. Sci. USA, 110, 20633–20638, https://doi.org/10.1073/pnas.1309345110, 2013.
Azam, F., Fenchel, T., Field, J. G., Gray, J. S., Meyer-Reil, L. A., and Thingstad, F.: The Ecological Role of Water-Column Microbes in the Sea, Mar. Ecol.-Prog. Ser., 10, 257–263, https://doi.org/10.3354/meps010257, 1983.
Bach, L. T., Riebesell, U., and Schulz, K. G.: Distinguishing between the effects of ocean acidification and ocean carbonation in the coccolithophore Emiliania huxleyi, Limnol. Oceanogr., 56, 2040–2050, https://doi.org/10.4319/lo.2011.56.6.2040, 2011.
Bach, L. T., Riebesell, U., Gutowska, M. A., Federwisch, L., and Schulz, K. G.: A unifying concept of coccolithophore sensitivity to changing carbonate chemistry embedded in an ecological framework, Prog. Oceanogr., 135, 125–138, https://doi.org/10.1016/j.pocean.2015.04.012, 2015.
Bach, L. T., Alvarez-Fernandez, S., Hornick, T., Stuhr, A., and Riebesell, U.: Simulated ocean acidification reveals winners and losers in coastal phytoplankton, PLoS One, 12, e0188198, https://doi.org/10.1371/journal.pone.0188198, 2017.
Bach, L. T., Hernández-hernández, N., Taucher, J., Spisla, C., Sforna, C., Riebesell, U., and Aristegui, J.: Effects of Elevated CO2 on a Natural Diatom Community in the Subtropical NE Atlantic, Front. Mar. Sci., 6, 1–16, https://doi.org/10.3389/fmars.2019.00075, 2019.
Battarbee, R. W., Charles, D. F., Bigler, C., Cumming, B. F., and Renberg, I.: Diatoms as indicators as surface-water acidity, in Diatoms: Applications for the Environmental and Earth Sciences, edited by: Smol, J. P. and Stoermer, E. F., Cambridge University Press, Cambridge, 98–121, 2010.
Biswas, H., Cros, A., Yadav, K., Ramana, V. V., Prasad, V. R., Acharyya, T., and Babu, P. V. R.: The response of a natural phytoplankton community from the Godavari River Estuary to increasing CO2 concentration during the pre-monsoon period, J. Exp. Mar. Bio. Ecol., 407, 284–293, https://doi.org/10.1016/j.jembe.2011.06.027, 2011.
Biswas, H., Shaik, A. U. R., Bandyopadhyay, D., and Chowdhury, N.: CO2 induced growth response in a diatom dominated phytoplankton community from SW Bay of Bengal coastal water, Estuar. Coast. Shelf S., 198, 29–42, https://doi.org/10.1016/j.ecss.2017.07.022, 2017.
Boyd, P. and Newton, P.: Evidence of the potential influence of planktonic community structure on the interannual variability of particulate organic carbon flux, Deep-Sea Res. Pt. I, 42, 619–639, 1995.
Boyd, P. W.: Diatom traits regulate Southern Ocean silica leakage, P. Natl. Acad. Sci. USA, 110, 20358–20359, https://doi.org/10.1073/pnas.1320327110, 2013.
Boyd, P. W., Collins, S., Dupont, S., Fabricius, K., Gattuso, J. P., Havenhand, J., Hutchins, D. A., Riebesell, U., Rintoul, M. S., Vichi, M., Biswas, H., Ciotti, A., Gao, K., Gehlen, M., Hurd, C. L., Kurihara, H., Mcgraw, C. M., Navarro, J. M., Nilsson, G. E., Passow, U., and Pörtner, H. O.: Experimental strategies to assess the biological ramifications of multiple drivers of global ocean change-A review, Glob. Change Biol., 24, 2239–2261, https://doi.org/10.1111/gcb.14102, 2018.
Brzezinski, M. A. and Nelson, D. M.: Chronic substrate limitation of silicic acid uptake rates in the western Sargasso Sea, Deep-Sea Res. Pt. II., 43, 437–453, https://doi.org/10.1016/0967-0645(95)00099-2, 1996.
Burkhardt, S., Amoroso, G., Riebesell, U., and Sueltemeyer, D.: CO2 and uptake in marine diatoms acclimated to different CO2 concentrations, Limnol. Oceanogr., 46, 1378–1391, 2001.
Calvo-Díaz, A., D́az-Pérez, L., Suárez, L. Á., Morán, X. A. G., Teira, E., and Marañón, E.: Decrease in the autotrophic-to-heterotrophic biomass ratio of picoplankton in oligotrophic marine waters due to bottle enclosure, Appl. Environ. Microb., 77, 5739–5746, https://doi.org/10.1128/AEM.00066-11, 2011.
Carpenter, S. R.: Microcosm Experiments Have Limited Relevance for Community and Ecosystem Ecology, Ecology, 77, 667–680, 1996.
Cripps, G., Flynn, K. J., and Lindeque, P. K.: Ocean acidification affects the phyto-zoo plankton trophic transfer efficiency, PLoS One, 11, 1–15, https://doi.org/10.1371/journal.pone.0151739, 2016.
Davidson, A., McKinlay, J., Westwood, K., Thomson, P., van den Enden, R., de Salas, M., Wright, S., Johnson, R., and Berry, K.: Enhanced CO2 concentrations change the structure of Antarctic marine microbial communities, Mar. Ecol.-Prog. Ser., 552, 93–113, https://doi.org/10.3354/meps11742, 2016.
Domingues, R. B., Guerra, C. C., Galvao, H. M., Brotas, V., and Barbosa, A. B.: Short-term interactive effects of ultraviolet radiation, carbon dioxide and nutrient enrichment on phytoplankton in a shallow coastal lagoon, Aquat. Ecol., 51, 91–105, https://doi.org/10.1007/s10452-016-9601-4, 2017.
Donahue, K., Klaas, C., Dillingham, P. W., and Hoffmann, L. J.: Combined effects of ocean acidification and increased light intensity on natural phytoplankton communities from two Southern Ocean water masses, J. Plankton Res., 41, 30–45, https://doi.org/10.1093/plankt/fby048, 2019.
Duarte, C. M., Gasol, J. M., and Vaqué, D.: Role of experimental approaches in marine microbial ecology, Aquat. Microb. Ecol., 13, 101–111, https://doi.org/10.3354/ame013101, 1997.
Duarte, C. M., Hendriks, I. E., Moore, T. S., Olsen, Y. S., Steckbauer, A., Ramajo, L., Carstensen, J., Trotter, J. A., and McCulloch, M.: Is Ocean Acidification an Open-Ocean Syndrome? Understanding Anthropogenic Impacts on Seawater pH, Estuar. Coast., 36, 221–236, https://doi.org/10.1007/s12237-013-9594-3, 2013.
Dutkiewicz, S., Morris, J. J., Follows, M. J., Scott, J., Levitan, O., Dyhrman, S. T., and Berman-Frank, I.: Impact of ocean acidification on the structure of future phytoplankton communities, Nat. Clim. Change, 5, 1002–1006, https://doi.org/10.1038/nclimate2722, 2015.
Eggers, S. L., Lewandowska, A. M., Barcelos e Ramos, J., Blanco-Ameijeiras, S., Gallo, F., and Matthiessen, B.: Community composition has greater impact on the functioning of marine phytoplankton communities than ocean acidification, Glob. Change Biol., 20, 713–723, https://doi.org/10.1111/gcb.12421, 2014.
Endo, H., Yoshimura, T., Kataoka, T., and Suzuki, K.: Effects of CO2 and iron availability on phytoplankton and eubacterial community compositions in the northwest subarctic Pacific, J. Exp. Mar. Biol. Ecol., 439, 160–175, https://doi.org/10.1016/j.jembe.2012.11.003, 2013.
Endo, H., Sugie, K., Yoshimura, T., and Suzuki, K.: Effects of CO2 and iron availability on rbcL gene expression in Bering Sea diatoms, Biogeosciences, 12, 2247–2259, https://doi.org/10.5194/bg-12-2247-2015, 2015.
Endo, H., Sugie, K., Yoshimura, T., and Suzuki, K.: Response of Spring Diatoms to CO2 Availability in the Western North Pacific as Determined by Next-Generation Sequencing, PLoS One, 11, e0154291, https://doi.org/10.1371/journal.pone.0154291, 2016.
Fabricius, K. E., Langdon, C., Uthicke, S., Humphrey, C., Noonan, S., De'ath, G., Okazaki, R., Muehllehner, N., Glas, M. S., and Lough, J. M.: Losers and winners in coral reefs acclimatized to elevated carbon dioxide concentrations, Nat. Clim. Change, 1, 165–169, https://doi.org/10.1038/nclimate1122, 2011.
Falkowski, P. G. and Raven, J. A.: Aquatic Photosynthesis, Princeton University Press, Princeton, 2007.
Feng, Y., Hare, C. E., Leblanc, K., Rose, J. M., Zhang, Y., DiTullio, G. R., Lee, P. A., Wilhelm, S. W., Rowe, J. M., Sun, J., Nemcek, N., Gueguen, C., Passow, U., Benner, I., Brown, C., and Hutchins, D. A.: Effects of increased pCO2 and temperature on the north atlantic spring bloom. I. The phytoplankton community and biogeochemical response, Mar. Ecol.-Prog. Ser., 388, 13–25, https://doi.org/10.3354/meps08133, 2009.
Feng, Y., Hare, C. E., Rose, J. M., Handy, S. M., DiTullio, G. R., Lee, P. A., Smith, W. O., Peloquin, J., Tozzi, S., Sun, J., Zhang, Y., Dunbar, R. B., Long, M. C., Sohst, B., Lohan, M., and Hutchins, D. A.: Interactive effects of iron, irradiance and CO2 on Ross Sea phytoplankton, Deep-Res. Pt. I, 57, 368–383, https://doi.org/10.1016/j.dsr.2009.10.013, 2010.
Ferguson, R. L., Buckley, E. N., and Palumbo, A. V.: Response of marine bacterioplankton to differential filtration and confinement, Appl. Environ. Microb., 47, 49–55, 1984.
Field, C. B., Behrenfeld, M. J., Randerson, J. T., and Falkowski, P. G.: Primary Production of the Biosphere: Integrating Terrestrial and Oceanic Components, Science, 281, 237–240, https://doi.org/10.1126/science.281.5374.237, 1998.
Flynn, K. J., Blackford, J. C., Baird, M. E., Raven, J. A., Clark, D. R., Beardall, J., Brownlee, C., Fabian, H., and Wheeler, G. L.: Changes in pH at the exterior surface of plankton with ocean acidification, Nat. Clim. Change, 2, 510–513, https://doi.org/10.1038/nclimate1489, 2012.
Fogg, G. E. and Calvario-Martinez, O.: Effects of bottle size in determinations of primary productivity by phytoplankton, Hydrobiologia, 173, 89–94, https://doi.org/10.1007/BF00015518, 1989.
Friedrichs, L., Hörnig, M., Schulze, L., Bertram, A., Jansen, S., and Hamm, C.: Size and biomechanic properties of diatom frustules influence food uptake by copepods, Mar. Ecol.-Prog. Ser., 481, 41–51, https://doi.org/10.3354/meps10227, 2013.
Gao, K. and Campbell, D. A.: Photophysiological responses of marine diatoms to elevated CO2 and decreased pH: A review, Funct. Plant Biol., 41, 449–459, https://doi.org/10.1071/FP13247, 2014.
Gao, K., Xu, J., Gao, G., Li, Y., Hutchins, D. A., Huang, B., Wang, L., Zheng, Y., Jin, P., Cai, X., Häder, D. P., Li, W., Xu, K., Liu, N., and Riebesell, U.: Rising CO2 and increased light exposure synergistically reduce marine primary productivity, Nat. Clim. Change, 2, 519–523, https://doi.org/10.1038/nclimate1507, 2012.
Gaylord, B., Kroeker, K. J., Sunday, J. M., Anderson, K. M., Barry, J. P., Brown, N. E., Connell, S. D., Dupont, S., Fabricius, K. E., Hall-Spencer, J. M., Klinger, T., Milazzo, M., Munday, P. L., Russell, B. D., Sanford, E., Schreiber, S. J., Thiyagarajan, V., Vaughan, M. L. H., Widdicombe, S., and Harley, C. D. G.: Ocean acidification through the lens of ecological theory, Ecology, 96, 3–15, 2015.
Gazeau, F., Sallon, A., Pitta, P., Tsiola, A., Maugendre, L., Giani, M., Celussi, M., Pedrotti, M. L., Marro, S., and Guieu, C.: Limited impact of ocean acidification on phytoplankton community structure and carbon export in an oligotrophic environment: Results from two short-term mesocosm studies in the Mediterranean Sea, Estuar. Coast. Shelf S., 186, 72–88, https://doi.org/10.1016/j.ecss.2016.11.016, 2017.
Giordano, M., Beardall, J., and Raven, J. A.: CO2 concentrating mechanisms in algae: mechanisms, environmental modulation, and evolution., Annu. Rev. Plant Biol., 56, 99–131, https://doi.org/10.1146/annurev.arplant.56.032604.144052, 2005.
Goldenberg, S. U., Nagelkerken, I., Marangon, E., Bonnet, A., and Camilo, M.: Ecological complexity buffers the impacts of future climate on marine animals Corresponding author Keywords, Nat. Clim. Change, 8, 229–233, https://doi.org/10.1038/s41558-018-0086-0, 2018.
Grear, J. S., Rynearson, T. A., Montalbano, A. L., Govenar, B., and Menden-Deuer, S.: pCO2 effects on species composition and growth of an estuarine phytoplankton community, Estuar. Coast. Shelf S., 190, 40–49, https://doi.org/10.1016/j.ecss.2017.03.016, 2017.
Guangao, L.: Different types of ecosystem experiments, in Enclosed Experimental Marine Ecosystems: A Review and Recommendations, edited by: Lalli, C. M., Springer-Verlag, New York, 7–20, 1990.
Guiry, M. D.: How many species of algae are there?, J. Phycol., 48, 1057–1063, https://doi.org/10.1111/j.1529-8817.2012.01222.x, 2012.
Hall-Spencer, J. M., Rodolfo-Metalpa, R., Martin, S., Ransome, E., Fine, M., Turner, S. M., Rowley, S. J., Tedesco, D., and Buia, M.-C.: Volcanic carbon dioxide vents show ecosystem effects of ocean acidification, Nature, 454, 96–99, https://doi.org/10.1038/nature07051, 2008.
Hama, T., Inoue, T., Suzuki, R., Kashiwazaki, H., Wada, S., Sasano, D., Kosugi, N., and Ishii, M.: Response of a phytoplankton community to nutrient addition under different CO2 and pH conditions, J. Oceanogr., 72, 207–223, https://doi.org/10.1007/s10872-015-0322-4, 2016.
Hamm, C. and Smetacek, V.: Armor: Why, when, and how, in Evolution of Phytoplankton, edited by: Falkowski, P. G. and Knoll, A. H., Elsevier, Boston, 311–332, 2007.
Hamm, C. E., Merkel, R., Springer, O., Jurkojc, P., Maiert, C., Prechtelt, K., and Smetacek, V.: Architecture and material properties of diatom shells provide effective mechanical protection, Nature, 421, 841–843, https://doi.org/10.1038/nature01416, 2003.
Hammes, F., Vital, M., and Egli, T.: Critical evaluation of the volumetric “bottle effect” on microbial batch growth, Appl. Environ. Microb., 76, 1278–1281, https://doi.org/10.1128/AEM.01914-09, 2010.
Hare, C. E., Leblanc, K., DiTullio, G. R., Kudela, R. M., Zhang, Y., Lee, P. A., Riseman, S., and Hutchins, D. A.: Consequences of increased temperature and CO2 for phytoplankton community structure in the Bering Sea, Mar. Ecol.-Prog. Ser., 352, 9–16, https://doi.org/10.3354/meps07182, 2007.
Hervé, V., Derr, J., Douady, S., Quinet, M., Moisan, L., and Lopez, P. J.: Multiparametric Analyses Reveal the pH-Dependence of Silicon Biomineralization in Diatoms, PLoS One, 7, e46722, https://doi.org/10.1371/journal.pone.0046722, 2012.
Hofmann, G. E., Smith, J. E., Johnson, K. S., Send, U., Levin, L. A., Micheli, F., Paytan, A., Price, N. N., Peterson, B., Takeshita, Y., Matson, P. G., de Crook, E., Kroeker, K. J., Gambi, M. C., Rivest, E. B., Frieder, C. A., Yu, P. C., and Martz, T. R.: High-frequency dynamics of ocean pH: A multi-ecosystem comparison, PLoS One, 6, e28983, https://doi.org/10.1371/journal.pone.0028983, 2011.
Hopkins, F. E., Turner, S. M., Nightingale, P. D., Steinke, M., Bakker, D., and Liss, P. S.: Ocean acidification and marine trace gas emissions, P. Natl. Acad. Sci. USA, 107, 760–765, https://doi.org/10.1073/pnas.0907163107, 2010.
Hoppe, C. J. M., Hassler, C. S., Payne, C. D., Tortell, P. D., Rost, B. R., and Trimborn, S.: Iron limitation modulates ocean acidification effects on Southern Ocean phytoplankton communities, PLoS One, 8, e79890, https://doi.org/10.1371/journal.pone.0079890, 2013.
Hoppe, C. J. M., Schuback, N., Semeniuk, D. M., Maldonado, M. T., and Rost, B.: Functional Redundancy Facilitates Resilience of Subarctic Phytoplankton Assemblages toward Ocean Acidification and High Irradiance, Front. Mar. Sci., 4, 229, https://doi.org/10.3389/fmars.2017.00229, 2017a.
Hoppe, C. J. M., Schuback, N., Semeniuk, D., Giesbrecht, K., Mol, J., Thomas, H., Maldonado, M. T., Rost, B., Varela, D. E., and Tortell, P. D.: Resistance of Arctic phytoplankton to ocean acidification and enhanced irradiance, Polar Biol., 41, 399–413, https://doi.org/10.1007/s00300-017-2186-0, 2017b.
Hussherr, R., Levasseur, M., Lizotte, M., Tremblay, J.-É., Mol, J., Thomas, H., Gosselin, M., Starr, M., Miller, L. A., Jarniková, T., Schuback, N., and Mucci, A.: Impact of ocean acidification on Arctic phytoplankton blooms and dimethyl sulfide concentration under simulated ice-free and under-ice conditions, Biogeosciences, 14, 2407–2427, https://doi.org/10.5194/bg-14-2407-2017, 2017.
James, R. K., Hepburn, C. D., Cornwall, C. E., McGraw, C. M., and Hurd, C. L.: Growth response of an early successional assemblage of coralline algae and benthic diatoms to ocean acidification, Mar. Biol., 161, 1687–1696, https://doi.org/10.1007/s00227-014-2453-3, 2014.
Johnson, V. R., Brownlee, C., Rickaby, R. E. M., Graziano, M., Milazzo, M., and Hall-Spencer, J. M.: Responses of marine benthic microalgae to elevated CO2, Mar. Biol., 160, 1813–1824, https://doi.org/10.1007/s00227-011-1840-2, 2011.
Kim, J.-M., Lee, K., Shin, K., Kang, J.-H., Lee, H.-W., Kim, M., Jang, P.-G., and Jang, M.-C.: The effect of seawater CO2 concentration on growth of a natural phytoplankton assemblage in a controlled mesocosm experiment, Limnol. Oceanogr., 51, 1629–1636, https://doi.org/10.4319/lo.2006.51.4.1629, 2006.
Kim, J. M., Lee, K., Yang, E. J., Shin, K., Noh, J. H., Park, K. T., Hyun, B., Jeong, H. J., Kim, J. H., Kim, K. Y., Kim, M., Kim, H. C., Jang, P. G., and Jang, M. C.: Enhanced production of oceanic dimethylsulfide resulting from CO2-induced grazing activity in a high CO2 world, Environ. Sci. Technol., 44, 8140–8143, https://doi.org/10.1021/es102028k, 2010.
Kottmeier, D. M., Rokitta, S. D., and Rost, B.: H+-driven increase in CO2 uptake and decrease in uptake explain coccolithophores' acclimation responses to ocean acidification, Limnol. Oceanogr., 61, 2045–2057, https://doi.org/10.1002/lno.10352, 2016.
Liu, H., Chen, M., Zhu, F., and Harrison, P. J.: Effect of Diatom Silica Content on Copepod Grazing, Growth and Reproduction, Front. Mar. Sci., 3, 1–7, https://doi.org/10.3389/fmars.2016.00089, 2016.
Loucaides, S., van Cappellen, P., Roubeix, V., Moriceau, B., and Ragueneau, O.: Controls on the Recycling and Preservation of Biogenic Silica from Biomineralization to Burial, Silicon, 4, 7–22, https://doi.org/10.1007/s12633-011-9092-9, 2012.
Mallozzi, A. J., Errera, R. M., Bargu, S., and Herrmann, A. D.: Impacts of elevated pCO2 on estuarine phytoplankton biomass and community structure in two biogeochemically distinct systems in Louisiana, USA, J. Exp. Mar. Biol. Ecol., 511, 28–39, https://doi.org/10.1016/j.jembe.2018.09.008, 2019.
Malviya, S., Scalco, E., Audic, S., Vincent, F., Veluchamy, A., Poulain, J., Wincker, P., Iudicone, D., de Vargas, C., Bittner, L., Zingone, A., and Bowler, C.: Insights into global diatom distribution and diversity in the world's ocean, P. Natl. Acad. Sci. USA, 113, E1516–E1525, https://doi.org/10.1073/pnas.1509523113, 2016.
Mann, D. G. and Vanormelingen, P.: An Inordinate Fondness? The Number, Distributions, and Origins of Diatom Species, J. Eukaryot. Microbiol., 60, 414–420, https://doi.org/10.1111/jeu.12047, 2013.
Martin-Jézéquel, V., Hildebrand, M., and Brzezinski, M. A.: Review Silicon Metabolism in Diatoms: Implications for Growth, J. Phycol., 36, 821–840, 2000.
Maugendre, L., Gattuso, J.-P., Louis, J., de Kluijver, A., Marro, S., Soetaert, K., and Gazeau, F.: Effect of ocean warming and acidification on a plankton community in the NW Mediterranean Sea, ICES J. Mar. Sci., 72, 1744–1755, https://doi.org/10.1093/icesjms/fsu161, 2015.
Mejía, L. M., Isensee, K., Méndez-Vicente, A., Pisonero, J., Shimizu, N., González, C., Monteleone, B., and Stoll, H.: B content and Si/C ratios from cultured diatoms (Thalassiosira pseudonana and Thalassiosira weissflogii): Relationship to seawater pH and diatom carbon acquisition, Geochim. Cosmochim. Ac., 123, 322–337, https://doi.org/10.1016/j.gca.2013.06.011, 2013.
Menzel, D. W. and Case, J.: Concept and Design: Controlled Ecosystem Pollution Experiment, B. Mar. Sci., 27, 1–7, 1977.
Meunier, C. L., Algueró-Muñiz, M., Horn, H. G., Lange, J. A. F., and Boersma, M.: Direct and indirect effects of near-future pCO2 levels on zooplankton dynamics, Mar. Freshwater Res., 68, 373–380, https://doi.org/10.1071/MF15296, 2017.
Milligan, A. J., Varela, D. E., Brzezinski, M. A., and Morel, F. M. M.: Dynamics of Silicon Metabolism and Silicon Isotopic Discrimination in a Marine Diatom as a Function of pCO2, Limnol. Oceanogr., 49, 322–329, 2004.
Nelson, D. M., Tréguer, P., Brzezinski, M. A., Leynaert, A., and Quéguiner, B.: Production and dissolution of biogenic silica in the ocean: Revised global estimates, comparison with regional data and relationship to biogenic sedimentation, Global Biogeochem. Cy., 9, 359–372, https://doi.org/10.1029/95GB01070, 1995.
Nielsen, L. T., Jakobsen, H. H., and Hansen, P. J.: High resilience of two coastal plankton communities to twenty-first century seawater acidification: Evidence from microcosm studies, Mar. Biol. Res., 6, 542–555, https://doi.org/10.1080/17451000903476941, 2010.
Nielsen, L. T., Hallegraeff, G. M., Wright, S. W., and Hansen, P. J.: Effects of experimental seawater acidification on an estuarine plankton community, Aquat. Microb. Ecol., 65, 271–285, https://doi.org/10.3354/ame01554, 2012.
Nogueira, P., Domingues, R. B., and Barbosa, A. B.: Are microcosm volume and sample pre-filtration relevant to evaluate phytoplankton growth?, J. Exp. Mar. Biol. Ecol., 461, 323–330, https://doi.org/10.1016/j.jembe.2014.09.006, 2014.
Pan, Y., Zhang, Y., Peng, Y., Zhao, Q., and Sun, S.: Increases of chamber height and base diameter have contrasting effects on grazing rate of two cladoceran species: Implications for microcosm studies, PLoS One, 10, 1–14, https://doi.org/10.1371/journal.pone.0135786, 2015.
Pančić, M. and Kiørboe, T.: Phytoplankton defence mechanisms: traits and trade-offs, Biol. Rev., 92, 1269–1303, https://doi.org/10.1111/brv.12395, 2018.
Park, K. T., Lee, K., Shin, K., Yang, E. J., Hyun, B., Kim, J. M., Noh, J. H., Kim, M., Kong, B., Choi, D. H., Choi, S. J., Jang, P. G., and Jeong, H. J.: Direct linkage between dimethyl sulfide production and microzooplankton grazing, resulting from prey composition change under high partial pressure of carbon dioxide conditions, Environ. Sci. Technol., 48, 4750–4756, https://doi.org/10.1021/es403351h, 2014.
Paul, A. J., Bach, L. T., Schulz, K.-G., Boxhammer, T., Czerny, J., Achterberg, E. P., Hellemann, D., Trense, Y., Nausch, M., Sswat, M., and Riebesell, U.: Effect of elevated CO2 on organic matter pools and fluxes in a summer Baltic Sea plankton community, Biogeosciences, 12, 6181–6203, https://doi.org/10.5194/bg-12-6181-2015, 2015.
Pomeroy, L. R.: The ocean food web – A changing paradigm, Bioscience, 24, 499–504, 1974.
Primeau, F. W., Holzer, M., and DeVries, T.: Southern Ocean nutrient trapping and the efficiency of the biological pump, J. Geophys. Res.-Ocean., 118, 2547–2564, https://doi.org/10.1002/jgrc.20181, 2013.
Raven, J. A., Giordano, M., Beardall, J., and Maberly, S. C.: Algal and aquatic plant carbon concentrating mechanisms in relation to environmental change, Photosynth. Res., 109, 281–96, https://doi.org/10.1007/s11120-011-9632-6, 2011.
Reul, A., Muñoz, M., Bautista, B., Neale, P. J., Sobrino, C., Mercado, J. M., Segovia, M., Salles, S., Kulk, G., León, P., van de Poll, W. H. D., Pérez, E., Buma, A., and Blanco, J. M.: Effect of CO2, nutrients and light on coastal plankton. III. Trophic cascade, size structure and composition, Aquat. Biol., 22, 59–76, https://doi.org/10.3354/ab00585, 2014.
Robinson, C. and Williams, P. J. le B.: Respiration and its measurement in surface marine waters, in Respiration in Aquatic Environments, edited by: Del Giorgio, P. A. and Williams, P. J. le B., Oxford University Press, Oxford, 147–180, 2005.
Roleda, M. Y., Cornwall, C. E., Feng, Y., McGraw, C. M., Smith, A. M., and Hurd, C. L.: Effect of ocean acidification and pH fluctuations on the growth and development of coralline algal recruits, and an associated benthic algal assemblage, PLoS One, 10, 1–19, https://doi.org/10.1371/journal.pone.0140394, 2015.
Rossoll, D., Sommer, U., and Winder, M.: Community interactions dampen acidification effects in a coastal plankton system, Mar. Ecol.-Prog. Ser., 486, 37–46, https://doi.org/10.3354/meps10352, 2013.
Rost, B., Riebesell, U., Burkhardt, S., and Sültemeyer, D.: Carbon acquisition of bloom-forming marine phytoplankton, Limnol. Oceanogr., 48, 55–67, 2003.
Sala, M. M., Aparicio, F. L., Balagué, V., Boras, J. A., Borrull, E., Cardelús, C., Cros, L., Gomes, A., López-Sanz, A., Malits, A., Martínez, R. A., Mestre, M., Movilla, J., Sarmento, H., Vázquez-Domínguez, E., Vaqué, D., Pinhassi, J., Calbet, A., Calvo, E., Gasol, J. M., Pelejero, C., and Marrasé, C.: Contrasting effects of ocean acidification on the microbial food web under different trophic conditions, ICES J. Mar. Sci., 73, 670–679, https://doi.org/10.1093/icesjms/fsv130, 2015.
Sarmiento, J. L., Gruber, N., Brzezinski, M. A., and Dunne, J. P.: High-latitude controls of thermocline nutrients and low latitude biological productivity, Nature, 427, 56–60, https://doi.org/10.1038/nature10605, 2004.
Sarnelle, O.: Daphnia effects on microzooplankton: comparisons of enclosure and whole-lake responses, Ecology, 78, 913–928, https://doi.org/10.1016/S0010-4655(02)00300-4, 1997.
Sarthou, G., Timmermans, K. R., Blain, S., and Tréguer, P.: Growth physiology and fate of diatoms in the ocean: A review, J. Sea Res., 53, 25–42, https://doi.org/10.1016/j.seares.2004.01.007, 2005.
Schulz, K. G., Riebesell, U., Bellerby, R. G. J., Biswas, H., Meyerhöfer, M., Müller, M. N., Egge, J. K., Nejstgaard, J. C., Neill, C., Wohlers, J., and Zöllner, E.: Build-up and decline of organic matter during PeECE III, Biogeosciences, 5, 707–718, https://doi.org/10.5194/bg-5-707-2008, 2008.
Schulz, K. G., Bellerby, R. G. J., Brussaard, C. P. D., Büdenbender, J., Czerny, J., Engel, A., Fischer, M., Koch-Klavsen, S., Krug, S. A., Lischka, S., Ludwig, A., Meyerhöfer, M., Nondal, G., Silyakova, A., Stuhr, A., and Riebesell, U.: Temporal biomass dynamics of an Arctic plankton bloom in response to increasing levels of atmospheric carbon dioxide, Biogeosciences, 10, 161–180, https://doi.org/10.5194/bg-10-161-2013, 2013.
Schulz, K. G., Bach, L. T., Bellerby, R., Bermudez, R., Boxhammer, T., Czerny, J., Engel, A., Ludwig, A., Larsen, A., Paul, A., Sswat, M., and Riebesell, U.: Phytoplankton blooms at increasing levels of atmospheric carbon dioxide: experimental evidence for negative effects on prymnesiophytes and positive on small picoeukaryotes, Front. Mar. Sci., 4, 1–18, https://doi.org/10.3389/fmars.2017.00064, 2017.
Segovia, M., Lorenzo, M., Maldonado, M., Larsen, A., Berger, S., Tsagaraki, T., Lázaro, F., Iñiguez, C., García-Gómez, C., Palma, A., Mausz, M., Gordillo, F., Fernández, J., Ray, J., and Egge, J.: Iron availability modulates the effects of future CO2 levels within the marine planktonic food web, Mar. Ecol.-Prog. Ser., 565, 17–33, https://doi.org/10.3354/meps12025, 2017.
Sett, S., Schulz, K. G., Bach, L. T., and Riebesell, U.: Shift towards larger diatoms in a natural phytoplankton assemblage under combined high-CO2 and warming conditions, J. Plankton Res., 40, 391–406, https://doi.org/10.1093/plankt/fby018, 2018.
Shaik, A. U. R., Biswas, H., and Pal, S.: Increased CO2 availability promotes growth of a tropical coastal diatom assemblage (Goa coast, Arabian Sea, India), Diatom Res., 32, 325–339, https://doi.org/10.1080/0269249X.2017.1379443, 2017.
Shen, C. and Hopkinson, B. M.: Size scaling of extracellular carbonic anhydrase activity in centric marine diatoms, J. Phycol., 51, 255–263, https://doi.org/10.1111/jpy.12269, 2015.
Smetacek, V., Klaas, C., Strass, V. H., Assmy, P., Montresor, M., Cisewski, B., Savoye, N., Webb, A., d'Ovidio, F., Arrieta, J. M., Bathmann, U., Bellerby, R., Berg, G. M., Croot, P., Gonzalez, S., Henjes, J., Herndl, G. J., Hoffmann, L. J., Leach, H., Losch, M., Mills, M. M., Neill, C., Peeken, I., Röttgers, R., Sachs, O., Sauter, E., Schmidt, M. M., Schwarz, J., Terbrüggen, A., and Wolf-Gladrow, D.: Deep carbon export from a Southern Ocean iron-fertilized diatom bloom, Nature, 487, 313–319, https://doi.org/10.1038/nature11229, 2012.
Smetacek, V. S.: Role of sinking in diatom life-hystory: ecological, evolutionary and geological significance, Mar. Biol., 84, 239–251, https://doi.org/10.1007/BF00392493, 1985.
Sommer, U., Stibor, H., Katechakis, A., Sommer, F., and Hansen, T.: Pelagic food web confgurations at different levels of nutrient richness and their implications for the ratio fish production:primary production, Hydrobiologia, 484, 11–20, https://doi.org/10.1023/A:1021340601986, 2002.
Sommer, U., Paul, C., and Moustaka-Gouni, M.: Warming and ocean acidification effects on phytoplankton – From species shifts to size shifts within species in a mesocosm experiment, PLoS One, 10, 1–17, https://doi.org/10.1371/journal.pone.0125239, 2015.
Sournia, A., Chrétiennot-Dinet, M. J., and Ricard, M.: Marine phytoplankton: How many species in the world ocean?, J. Plankton Res., 13, 1093–1099, https://doi.org/10.1093/plankt/13.5.1093, 1991.
Spencer, M. and Warren, P. H.: The effects of habitat size and productivity on food web structure in small aquatic microcosms, Oikos, 75, 419–430, 1996.
Sswat, M., Stiasny, M., Taucher, J., Algueró-Muñiz, M., Bach, L. T., Jutfelt, F., Riebesell, U., and Clemmesen, C.: Food web changes under ocean acidification promote herring larvae survival, Nature Ecology and Evolution, 2, 836–840, 2018.
Tatters, A. O., Roleda, M. Y., Schnetzer, A., Fu, F., Hurd, C. L., Boyd, P. W., Caron, D. A., Lie, A. A. Y., Hoffmann, L. J., and Hutchins, D. A.: Short- and long-term conditioning of a temperate marine diatom community to acidification and warming, Philos. T. R. Soc. B, 368, 20120437, https://doi.org/10.1098/rstb.2012.0437, 2013.
Tatters, A. O., Schnetzer, A., Xu, K., Walworth, N. G., Fu, F., Spackeen, J. L., Sipler, R. E., Bertrand, E. M., McQuaid, J. B., Allen, A. E., Bronk, D. A., Gao, K., Sun, J., Caron, D. A., and Hutchins, D. A.: Interactive effects of temperature, CO2 and nitrogen source on a coastal California diatom assemblage, J. Plankton Res., 40, 151–164, https://doi.org/10.1093/plankt/fbx074, 2018.
Taucher, J., Jones, J., James, A., Brzezinski, M. A., Carlson, C. A., Riebesell, U., and Passow, U.: Combined effects of CO2 and temperature on carbon uptake and partitioning by the marine diatoms Thalassiosira weissflogii and Dactyliosolen fragilissimus, Limnol. Oceanogr., 60, 901–919, https://doi.org/10.1002/lno.10063, 2015.
Taucher, J., Arístegui, J., Bach, L. T., Guan, W., Montero, M. F., Nauendorf, A., Achterberg, E. P., and Riebesell, U.: Response of Subtropical Phytoplankton Communities to Ocean Acidification Under Oligotrophic Conditions and During Nutrient Fertilization, Front. Mar. Sci., 5, 1–14, https://doi.org/10.3389/fmars.2018.00330, 2018.
Thoisen, C., Riisgaard, K., Lundholm, N., Nielsen, T. G., and Hansen, P. J.: Effect of acidification on an Arctic phytoplankton community from Disko Bay, West Greenland, Mar. Ecol.-Prog. Ser., 520, 21–34, https://doi.org/10.3354/meps11123, 2015.
Thor, P. and Dupont, S.: Transgenerational effects alleviate severe fecundity loss during ocean acidification in a ubiquitous planktonic copepod, Glob. Change Biol., 21, 2261–2271, https://doi.org/10.1111/gcb.12815, 2015.
Thor, P. and Oliva, E. O.: Ocean acidification elicits different energetic responses in an Arctic and a boreal population of the copepod Pseudocalanus acuspes, Mar. Biol., 162, 799–807, https://doi.org/10.1007/s00227-015-2625-9, 2015.
Tortell, P. D., DiTullio, G. R., Sigman, D. M., and Morel, F. M. M.: CO2 effects on taxonomic composition and nutrient utilization in an Equatorial Pacific phytoplankton assemblage, Mar. Ecol.-Prog. Ser., 236, 37–43, https://doi.org/10.3354/meps236037, 2002.
Tortell, P. D., Payne, C. D., Li, Y., Trimborn, S., Rost, B., Smith, W. O., Riesselman, C., Dunbar, R. B., Sedwick, P., and DiTullio, G. R.: CO2 sensitivity of Southern Ocean phytoplankton, Geophys. Res. Lett., 35, L04605, https://doi.org/10.1029/2007GL032583, 2008.
Tréguer, P., Bowler, C., Moriceau, B., Dutkiewicz, S., Gehlen, M., Aumont, O., Bittner, L., Dugdale, R., Finkel, Z., Iudicone, D., Jahn, O., Guidi, L., Lasbleiz, M., Leblanc, K., Levy, M., and Pondaven, P.: Influence of diatom diversity on the ocean biological carbon pump, Nat. Geosci., 11, 27–37, https://doi.org/10.1038/s41561-017-0028-x, 2018.
Tréguer, P. J. and De La Rocha, C. L.: The World Ocean Silica Cycle, Annu. Rev. Mar. Sci., 5, 477–501, https://doi.org/10.1146/annurev-marine-121211-172346, 2013.
Trimborn, S., Lundholm, N., Thoms, S., Richter, K.-U., Krock, B., Hansen, P. J., and Rost, B.: Inorganic carbon acquisition in potentially toxic and non-toxic diatoms: the effect of pH-induced changes in seawater carbonate chemistry., Physiol. Plant., 133, 92–105, https://doi.org/10.1111/j.1399-3054.2007.01038.x, 2008.
Trimborn, S., Brenneis, T., Hoppe, C. J. M., Laglera, L. M., Norman, L., Santos-Echeandía, J., Völkner, C., Wolf-Gladrow, D., and Hassler, C. S.: Iron sources alter the response of Southern Ocean phytoplankton to ocean acidification, Mar. Ecol.-Prog. Ser., 578, 35–50, https://doi.org/10.3354/meps12250, 2017.
Vrieling, E. G., Gieskes, W. W. C., and Beelen, T. P. M.: SILICON DEPOSITION IN DIATOMS: CONTROL BY THE pH INSIDE THE SILICON DEPOSITION VESICLE, J. Phycol., 35, 548–559, https://doi.org/10.1046/j.1529-8817.1999.3530548.x, 1999.
Ward, B. A., Dutkiewicz, S., Jahn, O., and Follows, M. J.: A size-structured food-web model for the global ocean, Limnol. Oceanogr., 57, 1877–1891, https://doi.org/10.4319/lo.2012.57.6.1877, 2012.
Wilken, S., Hoffmann, B., Hersch, N., Kirchgessner, N., Dieluweit, S., Rubner, W., Hoffmann, L. J., Merkel, R., and Peeken, I.: Diatom frustules show increased mechanical strength and altered valve morphology under iron limitation, Limnol. Oceanogr., 56, 1399–1410, https://doi.org/10.4319/lo.2011.56.4.1399, 2011.
Witt, V., Wild, C., Anthony, K. R. N., Diaz-Pulido, G., and Uthicke, S.: Effects of ocean acidification on microbial community composition of, and oxygen fluxes through, biofilms from the Great Barrier Reef, Environ. Microbiol., 13, 2976–2989, https://doi.org/10.1111/j.1462-2920.2011.02571.x, 2011.
Wolf-Gladrow, D. and Riebesell, U.: Diffusion and reactions in the vicinity of plankton: A refined model for inorganic carbon transport, Mar. Chem., 59, 17–34, https://doi.org/10.1016/S0304-4203(97)00069-8, 1997.
Wolf, K. K. E., Hoppe, C. J. M., and Rost, B.: Resilience by diversity: Large intraspecific differences in climate change responses of an Arctic diatom, Limnol. Oceanogr., 63, 397–411, https://doi.org/10.1002/lno.10639, 2018.
Wu, Y., Campbell, D. A., Irwin, A. J., Suggett, D. J., and Finkel, Z. V.: Ocean acidification enhances the growth rate of larger diatoms, Limnol. Oceanogr., 59, 1027–1034, https://doi.org/10.4319/lo.2014.59.3.1027, 2014.
Yoshimura, T., Nishioka, J., Suzuki, K., Hattori, H., Kiyosawa, H., and Watanabe, Y. W.: Impacts of elevated CO2 on organic carbon dynamics in nutrient depleted Okhotsk Sea surface waters, J. Exp. Mar. Biol. Ecol., 395, 191–198, https://doi.org/10.1016/j.jembe.2010.09.001, 2010.
Yoshimura, T., Suzuki, K., Kiyosawa, H., Ono, T., Hattori, H., Kuma, K., and Nishioka, J.: Impacts of elevated CO2on particulate and dissolved organic matter production: Microcosm experiments using iron-deficient plankton communities in open subarctic waters, J. Oceanogr., 69, 601–618, https://doi.org/10.1007/s10872-013-0196-2, 2013.
Young, J. N., Kranz, S. A., Goldman, J. A. L., Tortell, P. D., and Morel, F. M. M.: Antarctic phytoplankton down-regulate their carbon-concentrating mechanisms under high CO2 with no change in growth rates, Mar. Ecol.-Prog. Ser., 532, 13–28, https://doi.org/10.3354/meps11336, 2015.
Zeebe, R. E. and Wolf-Gladrow, D. A.: CO2 in seawater: Equilibrium, kinetics, isotopes, Elsevier O., Elsevier, Amsterdam, 2001.